the Creative Commons Attribution 4.0 License.
the Creative Commons Attribution 4.0 License.
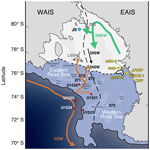
Return to the Ross Ice Shelf Project (RISP), Site J-9 (1977–1979): perspectives of West Antarctic Ice Sheet history from Miocene and Holocene benthic foraminifera
Serena N. Dameron
R. Mark Leckie
David Harwood
Reed Scherer
Peter-Noel Webb
In 1977–1978 and 1978–1979, the Ross Ice Shelf Project (RISP) recovered sediments from beneath the largest ice shelf in Antarctica at Site J-9 (∼82° S, 168° W), ∼450 km from open marine waters at the calving front of the Ross Ice Shelf and 890 km from the South Pole, one of the southernmost sites for marine sediment recovery in Antarctica. One important finding was the discovery of an active macrofauna, including crustaceans and fish, sustained below the ice shelf far from open waters. The sediment has a thin, unconsolidated upper unit (up to 20 cm thick) and a texturally similar but compacted lower unit (>1 m thick) containing reworked early, middle, and late Miocene diatom and calcareous benthic foraminiferal assemblages. A probable post-Last Glacial Maximum (LGM) disconformity separates the upper unit containing a dominantly agglutinated foraminiferal assemblage, from the lower unit consisting mostly of reworked Miocene calcareous benthic species, including Trifarina fluens, Elphidium magellanicum, Globocassidulina subglobosa, Gyroidina sp., and Nonionella spp. The presence of the polar planktic foraminiferal species Neogloboquadrina pachyderma and the endemic Antarcticella antarctica supports the late Miocene diatom age for the matrix of the lower unit. The microfossil assemblages indicate periods of ice sheet collapse and open-water conditions south of Site J-9 during warm intervals of the early, middle, and late Miocene, including the Miocene Climatic Optimum (∼17–14.7 Ma), demonstrating the dynamic nature of the West Antarctic Ice Sheet (WAIS) and Ross Ice Shelf during the Neogene. The foraminiferal assemblage of the upper unit is unique to the Ross Sea and suggests the influence of a sub-ice-shelf water mass proximal to the retreating post-LGM grounding zone. This unique assemblage is strongly dominated by the bathyal, cold-water agglutinated genus Cyclammina.
- Article
(5995 KB) - Full-text XML
-
Supplement
(568 KB) - BibTeX
- EndNote
Ice shelves serve important roles in stabilizing larger ice sheets (De Angelis and Skvarca, 2003; DeConto and Pollard, 2016). The Ross Ice Shelf (RIS), the largest ice shelf in the world ( km2; not quite as large as Spain but larger than California) buttresses the outflow of large ice streams and outlet glaciers of the West Antarctic Ice Sheet (WAIS) and East Antarctic Ice Sheet (EAIS). However, rising global temperatures and relatively warm ocean penetrating onto the continental shelf pose a threat to the stability of the Ross Ice Shelf (Stewart et al., 2019). This instability could lead to massive ice loss, retreat, and eventual collapse of the WAIS, which is particularly vulnerable due to most of its ice sheet being grounded below sea level, unlike the EAIS. Such a collapse could significantly contribute to global sea level rise (DeConto and Pollard, 2016; Paxman, 2023).
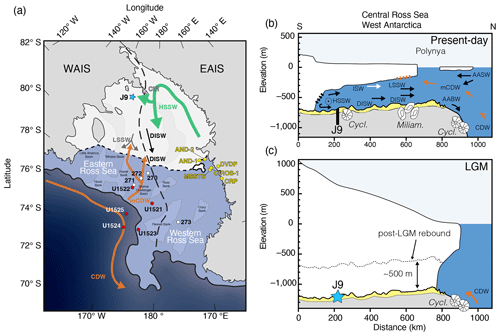
Figure 1Various views of the Ross Sea (modified from Tinto et al., 2019). (a) Map view of the Ross Sea oriented with the southern end on the top. The study location RISP J-9 is noted by a blue star, and previous drilling sites are indicated. Crary Ice Rise (CIR; gray circle), DSDP Leg 28 Sites (white circles), IODP Exp 374 Sites (red circles), and drilling projects in the western Ross Sea are indicated (yellow circles). The dashed black line marks the general provenance divide between sediments sourced from West Antarctica and East Antarctica. The thick black line defines the continental margin at 1000 m. (b) A south-to-north profile of the RIS depicting present-day conditions with water mass flow lines (see text for explanation). Cartoons of agglutinated benthic foraminifera depict Cyclammina trullissata at Site J-9 beneath the Ross Ice Shelf and on the continental slope of the eastern Ross Sea continental margin and Miliammina arenacea near the present-day calving front of the RIS (following Osterman and Kellogg, 1979). Site J-9 core is an exaggerated view of the maximum 122 cm drilled penetration depth. Gray color represents lower glacially derived unit and yellow is the post-LGM upper unit. (c) RIS during the LGM. The dotted line represents current position of seafloor before lithospheric depression of ∼500 m due to the grounded ice sheet load. LSSW stands for Low Salinity Shelf Water, mCDW stands for Modified Circumpolar Deep Water, DISW stands for Deep Ice Shelf Water, ISW stands for Ice Shelf Water, HSSW stands for High-Salinity Shelf Water, and AASW stands for Antarctic Surface Water.
The aim of this paper is to provide an overview of the marine paleoenvironment below the Ross Ice Shelf as recorded by fossil foraminifera that can offer insight into the stability of the WAIS during the Neogene. During two seasons (1977–1978 and 1978–1979), the Ross Ice Shelf Project (RISP) recovered sediments from Site J-9 (∼82° S) (Fig. 1) that date to the late Miocene based on diatom biostratigraphy (Brady and Martin, 1979; Harwood et al., 1989). Further study from RISP is important as it can provide a more comprehensive view of the evolution of the WAIS during the Miocene, particularly in the context of times of global warmth (e.g., Miocene Climatic Optimum, MCO, ∼17–14.7 Ma). Harwood et al. (1989) demonstrated that open marine conditions existed south of Site J-9 during episodes of the early Miocene, middle Miocene (e.g., MCO), and late Miocene based on the presence of distinct diatom assemblages in reworked diatomaceous clasts recovered in the RISP sediments and a mixture of highly fragmented diatoms of different ages in the sediment matrix. No diatoms of strictly Plio-Pleistocene age were noted by Harwood et al. (1989), which would indicate the addition of younger sediments from up-glacier sources (Scherer et al., 1998).
No detailed foraminiferal records have yet been published on the many gravity cores collected at Site J-9. The purpose of this contribution is to reassess the findings of an unpublished MS thesis (Greene, 1990) by re-examining the original processed RISP samples collected at Site J-9 (Webb, 1978, 1979), augmented by new analysis of archived original sediments. The primary goals of the study are to (1) provide relative age(s) of RISP samples; (2) document any evidence for reworking of older foraminifera; (3) compare foraminiferal data with the study of diatoms by Harwood et al. (1989); and (4) evaluate the foraminiferal assemblages in the context of paleoenvironment, including paleo-water depth and potential water mass affinity.
Sediment drilling and coring across the Ross Embayment provide a glacial history of the region including times when large ice sheets influenced the depositional record in the Ross Embayment. Large-scale continental ice sheets first appeared in East Antarctica at the Eocene–Oligocene transition (∼34 Ma) associated with decreasing temperatures and generally coinciding with the opening of the Southern Ocean gateways that thermally isolated the continent with the development of the Antarctic Circumpolar Current (e.g., Kennett, 1977, 1978; Zachos et al., 1992; Stickley et al., 2004; Coxall et al., 2005; Villa et al., 2014). A sharp drop in atmospheric pCO2 coupled with favorable orbital parameters likely triggered rapid glaciation of East Antarctica across the Eocene-Oligocene boundary based on a coupled global climate-ice sheet model (DeConto and Pollard, 2003; Galeotti et al., 2016). Terrestrial palynomorphs (Askin and Markgraf, 1986; Mildenhall, 1989; Askin and Raine, 2000; Raine and Askin, 2001; Prebble et al., 2006; Taviani et al., 2008; Warny et al., 2009; Sangiorgi et al., 2018) and TEX86 records from East Antarctica reveal the existence of warm conditions episodically during the late Oligocene and Neogene (Levy et al., 2016; Hartman et al., 2018; Sangiorgi et al., 2018; Duncan et al., 2022). High concentrations of dinoflagellate cysts recovered from AND-2A indicate peak warming ∼15.7 Ma during the Miocene Climatic Optimum (Warny et al., 2009).
The WAIS developed at a different rate than the EAIS (Uenzelmann-Neben et al., 2022). Earlier studies concluded that the WAIS did not fully develop over the Ross Embayment until the middle Miocene (Anderson and Bartek, 1992; De Santis et al., 1995; Chow and Bart, 2003; McKay et al., 2019), but recently Marschalek et al. (2021) provided evidence for the expansion of the WAIS across the continental shelf in the central Ross Sea during the late early Miocene (∼17.72–17.40 Ma). A series of facies sequences show that during colder periods of the Oligocene and early Miocene, the western Ross Sea experienced multiple glacial advance and retreat cycles (Leckie and Webb, 1983; Barrett et al., 1987; Webb, 1989; Fielding and Thomson, 1999; Fielding et al., 2000; Barrett, 2007; Kulhanek et al., 2019; Olivetti et al., 2023). Obliquity-paced ice sheet growth from the WAIS continued during the Miocene to Holocene (Barrett, 2007; Naish et al., 2009; Levy et al., 2019; Halberstadt et al., 2021, 2022). At its most extreme, the grounding line reached the continental shelf edge followed by more than 1000 km of retreat since the Last Glacial Maximum (LGM) to its present location (Kingslake et al., 2018; Anderson et al., 2014; Halberstadt et al., 2016; Bart et al., 2017; Greenwood et al., 2018). During interglacial periods, there was a switch from grounded ice to floating ice shelves to complete ice sheet collapse, resulting in open marine waters, depending on the magnitude and duration of warming.
Additionally, relatively warm surface waters have been documented to flow into cavities below ice shelves, tripling basal melting rates, particularly during austral summers (Jacobs et al., 1992; Hattermann et al., 2012; Pritchard et al., 2012; Stern et al., 2013; Holland et al., 2019; Stewart et al., 2019; Davison et al., 2023). This is expected to increase in the future with projected sea surface temperature increase and rising sea level, rendering WAIS particularly vulnerable since a large proportion of the grounding line is far below sea level (e.g., DeConto and Pollard, 2016; Gasson et al., 2016; Paxman, 2023). Findings from this study may have relevance for understanding the provenance of the sediments, including reworked foraminifera and other microfossils from West Antarctica via the Kamb Ice Stream, and the late Pleistocene–Holocene WAIS grounding zone history along the Siple Coast at drill site KIS-3 as part of the SWAIS2C drilling project (Patterson et al., 2022).
Site J-9 (82°22.5′ S, 168°37.5′ W) was located on the Ross Ice Shelf about 450 km from open marine waters at the calving front (Webb, 1978, 1979), and at the time it was one of the most southern locations of marine sediment recovery in Antarctica (Fig. 1a). It is located about 200 km away from the present-day grounding line along the Siple Coast (Kingslake et al., 2018). Reaching the seafloor required drilling an access hole through 420 m of floating ice and deploying sampling tools through the underlying 237 m of seawater to reach the mud line, 597 m below sea level (Fig. 2a). A flame-jet drill was used to penetrate through the ice during the 1977–1978 drilling season (Rand, 1977; Browning and Somerville, 1978; Clough and Hansen, 1979). Hot water drilling the following year was more efficient and effective (Browning et al., 1979; Koci, 1982). Two types of coring methods were used through the access hole that involved lowering gravity cores through the water column and dropping them at different heights above the mud line. A larger 22.5 cm diameter core barrel, known as a sphincter corer, was used to recover the water–sediment interface. This was done by dropping the core barrel 10–20 m above the seafloor and penetrating the sediment; a sphincter ring was rotated upon withdrawal, trapping the sediment in the core (Lipps et al., 1979). Weighted gravity corers penetrated further into the seafloor and were smaller, with diameters of 6.7 and 8.3 cm. They were allowed to free-fall at a greater distance, varying from 20 to 40 m above the seafloor. This method enabled penetration up to 122 cm into the seafloor (Webb, 1978, 1979).
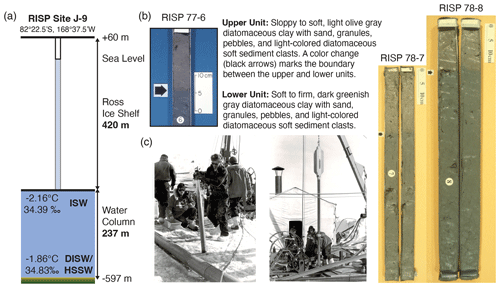
Figure 2Ross Ice Shelf Project (RISP) Site J-9, and representative cores and coring operations. (a) Schematic summary of Site J-9. Temperature and salinity measurements from Jacobs et al. (1979). ISW stands for Ice Shelf Water, and DISW stands for Deep Ice Shelf Water, which is derived from mixing with High-Salinity Shelf Water (HSSW) from the western Ross Sea. (b) Representative cores. The arrow marks the boundary between the lower and upper unit. Core 6 from the 1977–1978 season. Cores 7 and 8 from the 1978–1979 season. Sediment description from Webb (1978, 1979). (c) Two photographs from December 1978 coring operations. Geologists pictured are, left to right, Peter-N. Webb, Richard White, Howard Brady (holding core), and Mark Leckie. Photo credit: Peter-N. Webb.
Site J-9 was cored during two austral summer seasons of 1977–1978 and 1978–1979. The northward movement of the Ross Ice Shelf has been conservatively estimated to be ∼1 m d−1 , which means the distance between the 1977 and 1978 coring seasons is about ∼370 m. The first season recovered 11 gravity cores fitted with 2 m long core barrels, with the deepest penetration of 102 cm, and 10 sphincter cores with a maximum penetration of 14 cm (Webb, 1978). The second ∼3-week field season recovered 47 gravity cores up to 122 cm in length (Webb, 1979). Sediment penetration never exceeded core barrel length, despite attempting different free-fall distances above the seafloor. Field reports from the 1977/78 and 1978/79 drilling seasons provide a table of drilling dates and start time for each core, with each drilling season lasting 2–3 weeks. Provided at least a 1 m d−1 (or 0.42 m h−1) northward movement of the ice shelf and time interval between each drilled core, a rough estimate of distance between cores can be calculated. For example, up to eight cores over a 9 h window were recovered, providing a tight transect between cores from as low as tens of centimeters apart.
3.1 Lithology
The cored sediments are divided into an upper and lower unit based on a thin (<1 cm) brown orange iron-rich boundary that occurs anywhere between ∼4 to 20 cm depth (Fig. 2b). This sharp color contact is thought to be due to chemical alteration as opposed to sedimentary in origin since the clasts have the same composition in both units (Webb, 1978; Wrenn and Beckman, 1982; Raiswell and Tan, 1985). Raiswell and Tan (1985) proposed that a discontinuity in sedimentation caused an oxidation of sulfidic sediments exposed on the seafloor as they interacted with oxygenated seawater. Both units are described as an olive gray diamict of diatomaceous sandy mud with metamorphic and igneous clasts and soft sediment clasts. The main difference between the units is that the upper section is soft to soupy and is a lighter olive gray color with iron micronodules scattered throughout. The lower unit is distinctly firmer and darker in color. Angular to subrounded and glacially facetted pebbles, granules, and coarse sand-sized grains of granite, gneiss, and schist appear throughout the upper and lower units. The mineral composition and age of metamorphic and igneous grains are consistent with Marie Byrd Land or Whitmore Mountain provenance (Webb, 1978, 1979; Webb et al., 1979), south of major WAIS ice streams that flow into the Ross Sea. A big difference exists in the composition of smear slides between sediment clasts and the diamict matrix. The light tan-colored sediment clasts are semi-indurated, consisting of 73 %–92 % diatoms, whereas smear slides of the diamict matrix consist of ∼40 % diatoms, 30 % quartz, and 25 % clay. The diamict matrix consists of 5 % pebbles–granules, ∼21 % sand, and ∼74 % silty clay that was deposited either from floating ice or basal till (Webb, 1979; Harwood and Scherer, 1988; Harwood et al., 1989). The lack of any stratification suggests the latter.
The biogenic composition of the core consists predominantly of diatoms that are poorly to well preserved, with moderately high fragmentation consistent with in situ diatomite compaction, rather than subglacial shearing (Scherer et al., 2004). The dominant sediment clasts are derived from lower Miocene strata, consisting of a diatom-rich matrix. However, the matrix of both upper and lower units also includes rare middle and upper Miocene and Paleogene diatoms. The lack of a younger component is interpreted as reflecting erosion and transport during prior grounding line advances (Sjunneskog and Scherer, 2005). Diatom fragmentation is higher in the upper unit than the lower unit (Webb, 1978, 1979; Brady and Martin, 1979; Harwood et al., 1989), possibly reflecting subglacial processes. Silicoflagellates, radiolaria, foraminifera, sponge spicules, ebridians, spores, and palynomorphs are also present in smear slide analyses throughout the recovered material but are much rarer (Webb, 1979).
3.2 Previous foraminiferal results
Foraminiferal analyses of RISP samples were originally performed by Greene (1990), who analyzed 21 samples from the upper unit and 77 samples from the lower unit from cores collected during the two seasons. Sample volumes varied from core catcher samples having the largest volume of material, ∼500 CC of residue, sphincter core samples with ∼200 CC of residue, and interval sections having a much smaller volume of ∼10 CC of material for every 2 cm of sampled core. Greene (1990) processed samples by soaking and disaggregating the residue in a 5 % Calgon solution for up to 48 h. The disaggregated samples were washed over a series of stacked sieves (63, 125, 250, and 500 µm size) and then oven-dried at 100 °C. A floating technique was used to separate foraminifera from denser material by pouring the dried sample in a heavy liquid solution of carbon tetrachloride. The floated material was examined, and all foraminifera were picked. The upper unit yielded an average of 41 specimens per sample with the highest total foraminiferal count of 216 specimens in a sample; the lower unit averaged 9 specimens with a highest count of 63 foraminifera in a sample. Picked foraminifera were mounted on assemblage slides. The results of Greene's (1990) thesis were never published, and picked slides were not available for this foraminiferal review.
Only 21 of the 77 samples examined by Greene (1990) from the lower unit contain foraminifera (27.3 %; 445 specimens total in 21 samples). The assemblage is overwhelmingly dominated by perforate calcareous benthic species with a total of 41 species reported from the lower unit. Foraminiferal abundances in the lower unit are very low; 56 of 77 samples were barren (72.7 %) and the largest assemblage, from a ∼200 CC core catcher sample, has only 63 specimens. Greene reports that preservation is good to excellent in the lower unit, suggesting that carbonate dissolution is not the primary reason for the low foraminiferal abundances and barren samples.
The most abundant and persistent species reported by Greene (1990) from the lower unit are a uvigerinid identified as Trifarina fluens (152 specimens in 17 samples) and Elphidium magellanicum (58 specimens in 17 samples). Both of these taxa occur commonly in the upper Oligocene–lower Miocene of DSDP Site 270 (Leckie and Webb, 1983, 1986). A species of Uvigerina and E. magellanicum are also common in the middle Miocene of DSDP Site 273 (D'Agostino, 1980), Site 272 (Steinhauff, 1985), and IODP Site U1521 (Bombard et al., 2024). Secondary species in the lower unit of the RISP cores include Gyroidina sp. (30 specimens in 11 samples), Nonionella bradii (19 specimens in 6 samples), Globocassidulina subglobosa (18 specimens in 8 samples), Melonis barleeanus (14 specimens in 8 samples), Fissurina annectens (11 specimens in 7 samples), Nonionella iridea (10 specimens in 5 samples), Globobulimina auriculata (8 specimens in 4 samples), and Epistominella vitrea, reported as E. exigua (6 specimens in 6 samples). All these species are characteristic of the Miocene of the Ross Sea. The endemic Miocene planktic foraminiferal species Antarcticella antarctica (formerly Candeina antarctica; Leckie and Webb, 1985) occurs fairly consistently (24 specimens in 10 samples), while the late Miocene to recent polar species Neogloboquadrina pachyderma (8 specimens in 6 samples) provides a maximum age (∼11 Ma; Kucera and Schönfeld, 2007) for the lower unit. The presence of endemic benthic Ammoelphidiella uniforamina (3 samples) also supports a Miocene age for the lower unit (Leckie and Webb, 1986).
The thin upper unit is characterized by a completely different benthic foraminiferal assemblage dominated by agglutinated taxa. Foraminifera occur in 19 of the 22 upper-unit samples examined (86.4 %; 1061 specimens in 19 samples). The distinct upper-unit assemblage is restricted to the upper 13.5 cm according to Greene (1990). A total of 21 species are reported from the upper unit: 9 agglutinated species, 11 calcareous benthic, and 1 planktic species. Two agglutinated benthics dominate the upper-unit assemblage: Cyclammina trullissata (874 specimens in 17 samples) and Rhabdammina aff. R. abyssorum (118 specimens in 8 samples). Cyclammina sp. (24 specimens in 8 samples) is likely closely related to C. trullissata. Perforate calcareous benthics are very rare and include a variety of nodosariids (Dentalina, Fissurina, Lagena, Oolina, Parafissurina, Vaginulina). Very rare specimens of Elphidium magellanicum and Trifarina fluens are likely reworked from Miocene deposits. A single miliolid specimen (Quinqueloculina) was found in the upper unit. Rare planktic Neogloboquadrina pachyderma (7 specimens in 4 samples) was reported from the upper unit.
3.3 Great age debate
The microfossil assemblages are contained within reworked diamict (Webb, 1978, 1979) transported by ice from marine strata in the Ross Sea embayment or from an interior marine basin of some unknown distance and deposited during one of the many periods of glacial advance and retreat. The exception to this is the agglutinated and minor calcareous foraminifera in surface samples that are likely in situ and Holocene in age (Webb, 1978).
The various types of microfossils identified converge on a late Miocene age for the matrix of the RISP lower diamict unit. This is based on the youngest fossils identified in the matrix, although most of the diatoms in the matrix are of early Miocene age as a result of considerable reworking of older material (Harwood et al., 1989). This lower Miocene assemblage is uniquely identified by studying the abundant sediment clasts distributed throughout the matrix. The youngest diatoms identified in the matrix are of late Miocene age; are not found in clasts; and include Thalassiosira oliverana var. sparsa, Actinocyclus octonarius, and silicoflagellate Stephanocha pseudofibula. Spores and pollen range in age from the late Oligocene to Miocene (Brady and Martin, 1979; Jiang and Harwood, 1993) and are similar to the late Oligocene assemblage at DSDP Site 270 (Kemp and Barrett, 1975). Lower diversity and low abundance of Proteacidites spp. and lack of Myrtaceae at RISP suggest a Miocene instead of Oligocene age. The pollen assemblage may indicate a temperate forest, similar to what was found in the Cape Roberts Project drill core sediments on the western (McMurdo Sound) side of the Ross Sea (Askin and Raine, 2000). A larger ice cap in the late middle Miocene as compared to the Oligocene contributed to harsher conditions for vegetation growth and hence lower diversity (Brady and Martin, 1979). However, Wrenn and Beckman (1982) concluded that these pollen assemblages were likely reworked Paleogene flora. Silicoflagellates and ebridians were assigned an early–middle Miocene age (Ling and White, 1979). Initial assessments of calcareous benthic foraminifera in the lower unit are similar to lower- to mid-Miocene sediments from DSDP Leg 28 (Webb, 1978, 1979).
The diatom zonal schemes from drill sites in the Antarctic region (DSDP Leg 28, McCollum, 1975; DSDP Leg 29, Schrader, 1976) were utilized at RISP, where four distinct age ranges were determined: early Miocene, middle Miocene, late Miocene (Brady, 1978; Brady and Martin, 1979; Harwood and Scherer, 1988; Harwood et al., 1989), and controversially late Pleistocene age (Kellogg and Kellogg, 1980, 1981, 1983, 1986). This age controversy was settled by the careful work of Harwood et al. (1989), who were able to identify distinct ages of reworked assemblages by analyzing both sediment matrix and diatom-rich clasts. In addition, clearer taxonomic concepts and sieving the sediments to yield identifiable diatoms out of the abundant fragments helped resolve the disputed ages of RISP sediments. Harwood et al. (1989) did not identify any diatom species with age ranges restricted to the Plio-Pleistocene in either the lower or upper units. Moreover, cosmogenic concentrations of beryllium-10 indicate the cores must be pre-Quaternary (Wrenn and Beckmann, 1982). The absence of a strictly Pliocene and/or Pleistocene diatom assemblage, similar to nearby Crary Rise (Scherer et al., 1988) within the soft sediment clasts or the matrix indicates erosional truncation of the seafloor by a multitude of late Miocene to Pleistocene grounding events. However, Pleistocene age diatoms have been recovered from beneath grounded ice along the Siple Coast (Scherer, 1991; Scherer et al., 1998; Coenen et al., 2015).
Raiswell and Tan (1985) analyzed the nature of diagenesis of the RISP J-9 cores in hopes of solving the late Pleistocene–Holocene versus Miocene age controversy of the sediments. Their findings, based on organic carbon, organic nitrogen, and sulfur diagenesis, suggested a Holocene, in situ origin for the upper unit. They could not definitively conclude whether the lower unit was Miocene or also post-LGM in age.
The original samples examined by Greene (1990) were re-evaluated in this study. These samples were provided by Peter N. Webb, the principal investigator of the RISP Project. Greene employed a method to effectively separate foraminifera from the abundant terrigenous material by heavy liquid separation, suspending the sample in carbon tetrachloride. This causes foraminifera to float and denser lithic grains to sink. Because Greene's picked slides could not be located, we decided to look through the original floated source material to verify that all foraminifera were picked. We looked through the >63 µm size fraction of previously “sunk” and floated material. The floating technique Greene used was effective because only a few additional foraminiferal specimens were found among the samples. An additional 105 unprocessed samples obtained from Reed Scherer and the Marine and Geology Repository at Oregon State University (OSU-MGR) were also examined.
Scherer provided 57 unwashed samples from his previous diatom analyses that have a smaller volume, ranging from 5 to 15 cc. An additional 22 samples (∼20 cc) were provided by the OSU-MGR, from cores 9 and 10 collected during the 1977–1978 drilling season and cores 35 and 45 from the 1978–1979 drilling season, from which samples were taken every 10 cm throughout each core. These new samples were freeze-dried and then treated to five freeze–thaw treatments in a sodium sulfate solution, which is effective at safely breaking down indurated material (John Firth, personal communication, 2022).
OSU-MGR also provided the full archived halves of cores 7 and 8 (1978–1979), which are 78 and 84 cm in length, respectively. The entire core was processed, and all sediment material was examined thoroughly. Sampling of the upper unit was conducted every 2–3 cm to ascertain whether foraminifera were distributed uniformly or limited to the uppermost centimeters. The aim of this was to determine if the presence of foraminifera in the upper unit indicated a more recent age. Because data tables from Greene's thesis show that low-volume samples yield few foraminifera, samples in the lower unit were processed in ∼10 cm intervals (∼175 cc for samples from Core 7, and ∼250 cc for samples from the larger diameter Core 8). Samples were then washed over a 63 µm sieve and oven-dried at 50 °C.
Initially, all of the >125 µm size fraction for all new samples was examined, and only two picking trays were scanned of the 63–125 µm size fraction due to the larger amount of the finer fraction. Few foraminifera were noted in the small Scherer samples or OSU-MGR interval samples. We then floated the smaller size fraction <125 in a sodium polytungstate (SPT) heavy-liquid solution, which proved to be more effective than carbon-tetrachloride (Semensatto and Dias-Brito, 2007). This is also a safer method that does not require a fume hood and is non-carcinogenic. Sodium polytungstate has a density of 2.9 g mL−1. Calcite and quartz have similar densities of 2.7 and 2.65 g mL−1, respectively. However, trapped air in foraminiferal chambers likely make the tests more buoyant. The density of the SPT heavy liquid must be modified so that foraminiferal shells can be separated from denser lithic grains. A simple way to do this is to dilute SPT with distilled water until a piece of gypsum (2.32 g mL−1), a proxy for foraminiferal density, floats and a piece of orthoclase (2.57 g mL−1) sinks (Snyder and Huber, 1996). When the orthoclase sinks, the sample is poured directly into the SPT solution. Separation typically occurs within a few minutes, but as a precautionary measure, we would let the sample sit for about 15–20 min until we see no movement in the solution. Once the density separation is performed, the floated material is poured over filter paper and the remaining “sunk” portion is poured over another sheet of filter paper in which the sodium polytungstate solution is then dripped back into a beaker where it is reclaimed for future use (it is expensive). The two density fractions were each washed over a >63 µm sieve to remove the SPT and oven dried. Agglutinated foraminifera seem to float despite having a different composition, likely due to air pockets (Semensatto and Dias-Brito, 2007; this study).
We chose to assess the foraminiferal assemblages based on an aggregate view of the upper unit versus lower unit since the sediment recovered from the lower unit of RISP is all reworked marine biota. Foraminifera were identified at the species level and classified as planktic or benthic and calcareous or agglutinated.
Lastly, R-mode and Q-mode cluster analyses were performed on the foraminiferal dataset using the statistical software PAST (Hammer et al., 2001). A two-way paired group algorithm using the Bray–Curtis similarity index was applied to the abundance data to identify relationships among species and between samples. Only samples containing more than 20 specimens and a species abundance with a maximum abundance of at least 2 % were used in this analysis, consistent with other studies in the region (e.g., Bombard et al., 2024; Seidenstein et al., 2024) with the intention of identifying biofacies at RISP that could also be compared to other locations in the Ross Sea.
Sediment recovered in Site J-9 cores comprise mostly terrigenous and diatomaceous sediment. Foraminifera are very rare and of low diversity. Approximately 60 species were identified by Greene (1990) and are mostly sparsely distributed throughout the cores, except for a few dominant species that are characteristic of the upper and lower units (Table 1). A full list of all samples and taxa studied in this report and Greene's thesis can be found in Supplement Table S1. Unless otherwise stated, any discussion of foraminiferal results will be based on merging data from Greene's distribution table and newly processed samples processed in this study.
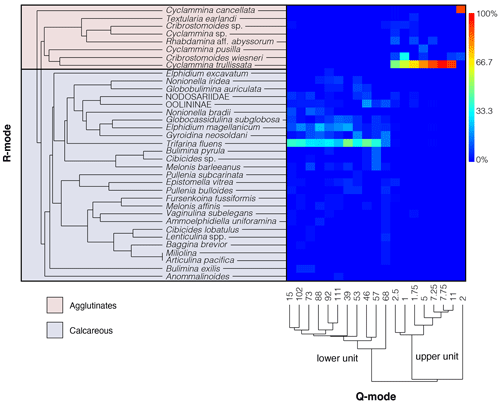
Figure 3R-mode and Q-mode cluster dendrograms of benthic foraminifera and a heatmap that shows the correlation between the two clustering methods. Warmer colors represent samples with high benthic foraminiferal abundance, while cooler colors indicate lower abundance. R-mode shows an agglutinated biofacies and calcareous biofacies. Q-mode cluster analysis shows two very distinct groups of an upper unit and lower unit. The heatmap clearly shows a very strong agglutinated Cyclammina-dominated assemblage in the upper unit, whereas the lower unit is composed almost exclusively of calcareous taxa, dominated by Trifarina fluens and secondarily by Elphidium magellanicum. Rarer species of Nodosaria, Dentalina, and Lagena have been grouped to Nodosariidae, and rarer species of Fissurina, Oolina, and Parafissurina have been grouped to Oolininae.
Distinct foraminiferal assemblage differences distinguish the upper and lower units: 99 % calcareous abundance in the lower unit and 92 % agglutinated abundance in the upper unit with rare calcareous taxa that were likely reworked from the lower unit (Figs. 3, 4). Upper-unit diversity ranges from 1–9 species per sample in samples containing foraminifera, while lower-unit diversity ranges from 1–21 species in samples containing foraminifera. Upper-unit samples have the greatest abundance, with up to 216 foraminiferal specimens, and 34 of the 67 samples had foraminifer recovery. The 33 samples that were barren come from interval samples with volumes between 5 to 20 CC, whereas most of the other samples come from the larger volume sphincter cores (Fig. 4; Greene, 1990; this study). The agglutinated assemblage is dominated by Cyclammina trullissata with ∼78.6 % of the total (cumulative) upper unit assemblage (Figs. 3, 4). Less common are Rhabdammina sp. aff. R. abyssorum (∼10.3 %, only observed by Greene), and rarer taxa () including Bathysiphon sp., Cribrostomoides wiesneri, Cyclammina cancellata and C. pusilla, Portatrochammina sp., and Textularia earlandi. Because we did not have access to the picked samples of Greene (1990), we could not confirm many of his identifications as we did not observe them in our samples. For example, in our analysis of upper-unit samples, we found no Rhabdammina, and we instead found many burrows that could be mistaken for the genus. We suspect this is the case for Greene. Neogloboquadrina pachyderma is the only planktic foraminifer that appears in the upper unit with a maximum of 4 specimens in one sample, 0–5 cm below the sediment–water interface (sphincter core sample RISP77/78-7, 0–5 cm, <1 % of the upper-unit assemblage).
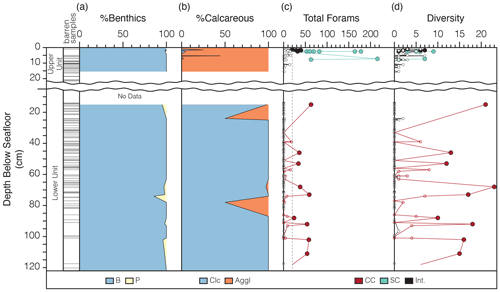
Figure 4Foraminiferal assessment of upper and lower units. Composite foraminiferal data from Greene (1990) and this study, arranged at an average sampled depth in the cores with barren samples noted along the depth axis. (a) The abundance of benthic foraminifera is shaded in blue with a small concentration of planktic foraminifera shown in yellow. (b) The calcareous abundance shown in blue with agglutinates in orange. (c) Total foraminifera and (d) total simple diversity within the cores are separated by sample type, where CC stands for core catcher samples (red, 500 CC), SC stands for sphincter core samples (green, 200 CC), and int indicates depth interval samples (black, ∼5–20 cc). A data gap (no samples) exists for the uppermost 20 cm of the lower unit section. Larger circles are samples that had 20 or more specimens and are included in the R-mode cluster analysis, while open circles contain less than 20 specimens and were excluded from the cluster analysis. Only core catcher (CC) samples from the lower unit contain enough specimens to be included in the cluster analysis (i.e., interval samples contain too few specimens). All sphincter core (SC) samples from the upper unit, and three interval samples from the uppermost sediments contain 20 or more specimens to be included in the R-mode cluster analysis. The wavy line indicates the disconformity between the upper and lower units.
Lower unit samples have a much lower foraminiferal abundance with a maximum of 61 specimens in one sample (core catcher sample from RISP 77, Core 11 at 15 cm depth), despite many of these low-specimen-yield samples coming from the larger volume core catcher samples (∼500 CC) as compared to the sphincter core samples (∼200 CC) in the upper unit. Samples from archived Cores 7 and 8 contain no foraminifera in the lower unit, which was surprising since the sampling volume was approximately the same as the sphincter cores and an order of magnitude larger than the interval samples. This suggests that dissolution of calcareous tests may have occurred within the sediments at some point while stored in the core repository.
Greene (1990) created a foraminiferal distribution table of only samples that yielded foraminifera (Table 1). We assume that samples not included were barren, which we corroborated by looking through the original source material. The majority of the barren samples come from interval sections (∼10 to 20 cm3), but 11 of 32 (∼500 CC) core catcher samples are also barren. The foraminiferal assemblage of the lower unit, in contrast to the upper unit, is composed almost exclusively of calcareous forms. Trifarina fluens is the most common species with an average 30 % abundance followed by Elphidium magellanicum that makes up an average 13 % abundance. Antarcticella antarctica, Fissurina annectens, Globocassidulina subglobosa, Gyroidina sp., Melonis barleeanus, Nonionella bradii, and Nonionella iridea have abundances between 2 %–6 %. The remaining calcareous taxa make up less than 2 % of the total (cumulative) lower unit assemblage. Two planktic foraminiferal species, Turborotalita quinqueloba and Neogloboquadrina pachyderma, are reported by Greene (1990) in the lower section and make up <1 % and 2 % average abundance, respectively. Cribrostomoides sp. is the only agglutinated taxa reported by Greene (1990) from the lower unit and is very rare, appearing in only three samples with one specimen per sample.
Table 1(a) Upper unit foraminiferal assemblage. (b) Lower unit foraminiferal assemblage. Foraminifera reported by Greene (1990) arranged by samples from the (a) upper unit and (b) lower unit of the RISP cores. (a) The upper unit shows 19 samples of the 22 samples examined contain foraminifera (13.6 % of the samples from the upper unit studied by Greene were barren). Rhabdammina are likely burrow fills. (b) The lower unit shows 21 samples of 77 examined contain foraminifera (72.7 % of the samples from the lower unit studied by Greene were barren). The majority of samples studied by Greene are barren. It is not known whether this a consequence of post-collection carbonate dissolution or very low foram abundances. We suspect that the low numbers of foraminifera from Greene's study are not the consequence of dissolution because of the very low concentration of organic matter in the RISP sediments and lack of gypsum in the stored cores. Primary species (P) are bolded, and accessory species (A) are indicated below each taxon. The numbers reported at the top of the highlighted columns record the number of samples containing each particular taxon out of the 19 samples from the upper unit or 21 samples from the lower unit that contain foraminifera. SC stands for sphincter core, CC stands for core catcher, and int indicates interval samples.
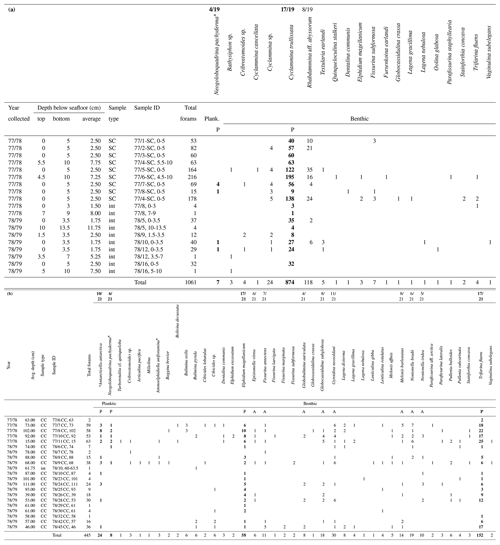
* Denotes age diagnostic species that support a late Miocene age for the lower unit.
6.1 Lower unit (upper Miocene)
Benthic foraminifera do not provide robust age control as taxa have long stratigraphic ranges. One workaround to this is to cross-reference the species at Site J-9 with those found elsewhere in the Ross Sea. By taking the maximum and minimum stratigraphic occurrences of those species, we get a more complete understanding of what the regional upper and lower age bounds are at RISP. The foraminiferal assemblage in this study is comprised of species whose occurrence at other localities range from the upper Oligocene to modern (Fig. 5; Osterman and Kellogg, 1979; D'Agostino, 1980; Steinhauff, 1985; Ishman and Webb, 1986; Leckie and Webb, 1986; Webb et al., 1986; Strong and Webb, 1998, 2001; Scherer et al., 1988; Scherer, 1991; Webb and Strong, 1998a, b, 2000; Galeotti and Coccioni, 1998; Galeotti et al., 2000; Patterson and Ishman, 2012; Majewski, 2013; Ridha et al., 2019; Seidenstein et al., 2024; Bombard et al., 2024).
The suspected planktic foraminiferal species Antarcticella antarctica is only known from the Ross Sea of Antarctica, including the upper Oligocene–lower Miocene of DSDP Site 270 (Leckie and Webb, 1980, 1983, 1985, 1986), Oligocene–lower Miocene of drill hole MSSTS-1 (Webb et al., 1986), upper Oligocene–lower Miocene of the CIROS-1 drill hole (Webb, 1989; Coccioni and Galeotti, 1997), lower to middle Miocene of DSDP Site 273 (D'Agostino and Webb, 1980; D'Agostino, 1980), middle Miocene of DSDP Site 272 (Steinhauff, 1985; Steinhauff and Webb, 1987), lower to middle Miocene of IODP Site U1521 (Bombard et al., 2024), and the upper Miocene of DVDP 10 and 11 (Ishman and Webb, 1986, 1988). This means that the foraminifera of the lower unit at RISP cannot be younger than ∼5.3 Ma (Miocene/Pliocene boundary). Likewise, the presence of Neogloboquadrina pachyderma, which first appears at or near the base of the Tortonian Stage (upper Miocene), constrains the lower unit to be no older than ∼11.8–9.6 Ma (Kucera and Schönfeld, 2007). The presence of the endemic Miocene benthic Ammoelphidiella uniforamina also helps to bracket a Miocene age for the lower unit. In summary, benthic and planktic foraminiferal assemblages from around the Ross Sea support a late Miocene age of the J-9 lower unit in agreement with diatom studies (i.e., Brady, 1979, 1983; Brady and Martin, 1979; Harwood and Scherer, 1988; Harwood et al., 1989).
Taking into consideration that RISP sediments have been reworked and transported some distance and likely deposited as glacial till in a glaciomarine setting, the diatom and foraminiferal assemblages can only provide a glimpse into the past. However, the presence of rare planktic foraminifera and dominance of diatoms support retreat and partial or complete collapse of the Ross Ice Shelf and West Antarctic Ice Sheet resulting in open marine conditions in the middle early Miocene, early middle Miocene, and middle late Miocene (Brady and Martin, 1979; Harwood and Scherer, 1988; Harwood et al., 1989). The absence of benthic diatoms supports depths exceeding the high latitude photic zone (>50 m), while paleo-depth estimates of the lower unit suggest neritic depths based on the relatively persistent occurrence of Elphidium magellanicum, Ammoelphidiella spp., and Nonionella spp. (e.g., Leckie and Webb, 1986; Murray, 1991; Hayward et al., 1999; Leckie and Olson, 2003).
Diagnostic Pliocene and Pleistocene diatoms that are found elsewhere in the Ross Sea are absent at Site J-9 (Harwood et al., 1989). There likely were Plio-Pleistocene microfossils at one time at the RISP site. For example, simulations of the WAIS during the Pleistocene predict favorable conditions for ice sheet collapse and open marine settings (Pollard and DeConto, 2009; DeConto et al., 2012). Sjunneskog and Scherer (2005) demonstrate from Ross Sea cores that glacial advance can erode, transport, and redeposit diatoms far from their source beds, and age-diagnostic Pleistocene marine diatoms occur in sediments further south along the Siple Coast supporting ice sheet collapse in the late Pleistocene (Scherer, 1991; Scherer et al., 1998). Subsequent advancing grounded ice sheets likely eroded Plio-Pleistocene sediment at RISP and transported it towards the continental shelf edge, leaving behind one of many such disconformities that are found across the Ross Sea (Sjunneskog and Scherer, 2005; Anderson and Bartek, 1992; McKay et al., 2019; Conte et al., 2021). The boundary between the upper and lower unit may very well be a composite of the most recent Ross Sea unconformities (RSU) noted at other drilling locations in the Ross Sea with little post-LGM sediment accumulation beneath the ice shelf (Houtz and Meijer, 1970; Houtz and Davey, 1973; Fillon, 1975; Hayes and Frakes, 1975; Savage and Ciesielski, 1983; Anderson and Bartek, 1992).
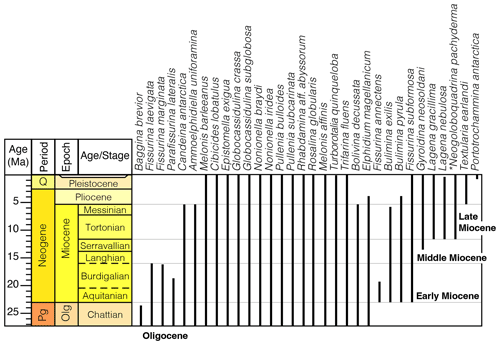
Figure 5Known stratigraphic ranges of key species reported at other locations in the Ross Sea and nearby regions that also appear at RISP (Kennett, 1968; Anderson, 1975; Osterman and Kellogg, 1979; D'Agostino, 1980; Leckie and Webb, 1983, 1985, 1986; Ishman and Webb, 1986; Steinhauff, 1985; Scherer et al., 1988; Scherer, 1991; Galeotti and Coccioni, 1998; Strong and Webb, 1998, 2001; Webb and Strong 1998a, b; Galeotti et al., 2000; Patterson and Ishman, 2012; Majewski, 2013; Ridha et al., 2019; Seidenstein et al., 2024).
6.2 Upper unit (Holocene)
The soft, soupy upper unit that overlies stiffer, more consolidated sediment further corroborates a younger depositional setting that has not been subjected to ice sheet loading, the last of which would have been the LGM (∼20 ka). This implies that the agglutinate-rich assemblage represents a more recent colonization of the seafloor sometime after the LGM. Reconstructions of the grounding line positions indicate this likely occurred after 7 ka when the grounding line was eastward from the vicinity of RISP (Anderson et al., 2014; Kingslake et al., 2018; Lowry et al., 2019; Neuhaus et al., 2021; Johnson et al., 2022). The thickness of the upper unit varies among the cores, with a thickness between 4 and 23 cm. Given the retreat of the grounding line from the RISP region ∼7 ka and assuming a constant accumulation rate, the sedimentation rate at RISP would be somewhere between 0.57–3.29 cm kyr−1. If this does represent a post-LGM assemblage, then a lack of Holocene diatoms in the samples suggests that the foraminifera lived under the ice shelf, which agrees with the low sedimentation rate. Further evidence of more recent foraminifer habitation comes from a closely spaced analysis (every 2–3 cm) of archived samples from the upper unit. The agglutinated assemblage is dominated by Cyclammina. The number of total foraminiferal specimens is greatest in the upper 2 cm with ∼81 % Cyclammina (46 % C. trullissata and 35 % C. cancellata) and 15 % Cribrostomoides wiesneri. The next 2–5 cm has no Cribrostomoides and is dominated by C. cancellata (82 %) and C. trullissata (18 %). These taxa decline sharply with increasing depth (Fig. 6). Most notably Cyclammina and other agglutinated foraminifera are absent in the lower unit. This suggests a completely different depositional setting for the upper unit. Because the upper unit developed beneath a retreating ice sheet and ice shelf, the presence of planktic foraminifera, diatoms, along with rarer calcareous taxa among an in situ agglutinated assemblage suggests these former microfossils were reworked from older sediments and derived from basal melt. The only other source for these foreign assemblages would be from open marine waters ∼450 km to the north, which is highly unlikely, as there would be evidence of extant species.
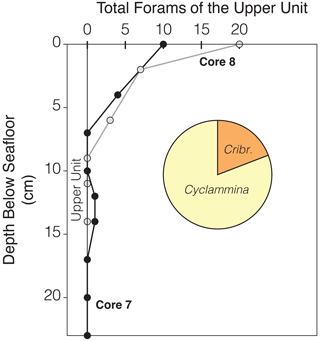
Figure 6An in-depth analysis of the upper unit on two archived cores (RISP78, cores 7 & 8) obtained from the Marine and Geology Repository at Oregon State University. The most abundant species are restricted to the uppermost 7 cm with rarer specimens found further down. The most dominant benthic foraminifera of the upper unit are shown in a pie diagram, consisting of agglutinated species of Cyclammina (81 % abundance; C. trullissata and C.cf. cancellata) and Cribrostomoides (15 % abundance; dominantly C. wiesneri and 4 % Cribrostomoides spp.).
The assemblage of the upper unit is unique to the Ross Sea with no known modern analog. The position of Site J-9 is about 200 km from the grounding line in a sub-ice shelf setting. The RISP assemblage is in stark contrast to the calcareous taxa found in the western Ross Sea in which Globocassidulina subglobosa is the most common taxon in the post-LGM calcareous foraminiferal assemblages, with Cassidulina neoteretis, Epistominella spp., Nonionella spp., Astrononion echolsi, Cibicides spp., and Trifarina earlandi as accessory taxa in distal sub-ice shelf and ice-proximal grounding zone settings (Melis and Salvi, 2009; Bart et al., 2016; Prothro et al., 2018; Smith et al., 2019; Majewski et al., 2020). Agglutinated taxa Miliammina arenacea and Portatrochammina spp. dominate under open marine conditions with diatom- and organic-rich sediments and/or in the presence of corrosive High-Salinity Shelf Water (HSSW; Melis and Salvin, 2009; Capotondi et al., 2018; Majewski et al., 2020).
The eastern Ross Sea shows similar assemblages, although Globocassidulina biora (smooth and pustulose forms) is the dominant calcareous benthic in the distal sub-ice-shelf and ice-proximal grounding zone facies (Majewski et al., 2018). Majewski et al. (2020) concluded that limited and episodic food supply in the western Ross Sea proximal grounding zone environment resulted in calcareous foraminiferal assemblages dominated by minute G. subglobosa in contrast to the larger specimens of G. biora that dominated the eastern Ross Sea proximal grounding zones. During the LGM, the grounding line was at or near the continental shelf edge of the eastern Ross Sea (Halberstadt et al., 2016). As the ice sheet retreated, a grounded zone wedge formed in the Whales Deep Basin forming a saddle between the Houtz Bank and Hayes Bank (McGlannan et al., 2017). After the LGM, calcareous taxa are rare within the Whales Deep Basin and agglutinated taxa Miliammina and Portatrochammina dominate. Majewski et al. (2018) attributed this to stagnant cold, saline waters, which are not conducive for calcite precipitation.
Hydrographic measurements show at least four unique water masses circulating in the Ross Sea (Fig. 7; Jacobs et al., 1979; Budillon et al., 2002, 2003, 2011; Orsi and Wiederwohl, 2009; Smith et al., 2012, 2019; Tinto et al., 2019). Relatively warm modified Circumpolar Deep Water (mCDW, −0.9 °C, S=34.5 ‰, 200–350 m) travels southward along western side of the Glomar Challenger Trough and enters below the Ross Ice Shelf in the central Ross Sea (Fig. 1a; Castagno et al., 2017). These waters also contribute to basal melting and correspond to the warm core water reported by Jacobs et al. (1978, 1979) along the ice shelf front. Models suggest mCDW only extends to about 100 km southward beneath the ice shelf (Tinto et al., 2019). High-Salinity Shelf Water (HSSW, −1.85 °C, S<34.7 ‰) forms from brine rejection during sea ice formation in the polynya of the western Ross Sea. Part of this HSSW flows to the north and over the continental break and contributes to Antarctic Bottom Water (AABW). Another branch of HSSW moves southward and flows beneath the Ross Ice Shelf along the base of the Transantarctic Mountains and contributes to basal melting along grounding lines. Simulations by Tinto et al. (2019) suggest that HSSW does not cross the provenance boundary between the East Antarctica and West Antarctica but instead travels northward at ∼170 °W (Fig. 1a). Temperature and salinity measurements at RISP (−1.86 °C, S=34.83 ‰; Jacobs et al., 1979) suggest that HSSW underlies Site J-9 (Fig. 7e; Clough and Hansen, 1979; Gilmour, 1979; Jacobs et al., 1978, 1979). Waters are stratified at RISP in which the temperature just below the base of the ice is −2.16 °C with a salinity of 34.39 ‰ (Fig. 2a; Jacobs et al., 1979). This corresponds to Ice Shelf Water (ISW; −2.03 °C, S=34.62 ‰, 350–500 m) that forms from fresh meltwater whose low salinity then moves northward below the ice, contributing to the very cold water mass temperature.
Warmer mCDW causes melting near the calving front of the Ross Ice Shelf forming another stratified layer of Low-Salinity Shelf Water (LSSW; −1.5 °C, S=34.5 ‰, 200–500 m). A concentration of DISW and LSSW moves out from beneath the ice shelf between 180 and 175 °W and into the Glomar Challenger Trough. LSSW spreads further towards the east, perhaps influenced by increased meltwater from Roosevelt Island (Jacobs et al., 1979; Budillon et al., 2003) with a northward plume between 170–165 °W in the Whales Deep Basin (Budillon et al., 2003). This is where Majewski et al. (2018) show a shift from calcareous distal sub-ice shelf assemblages with Globocassidulina subglobosa and G. biora to agglutinated Miliammina arenacea and Portatrochammina spp., which they attribute to colder, stagnant water and a shallower CCD. However, Osterman and Kellogg (1979) document a dominance of Miliammina arenacea (Fig. 7f, Assemblage 6) in a water depth of 500–800 m at the ice shelf edge and directly in front of a plume of LSSW and DISW (Fig. 7c, d). The extension of the LSSW in the Whales Deep Basin could be contributing to the assemblage reported by Majewski et al. (2018).
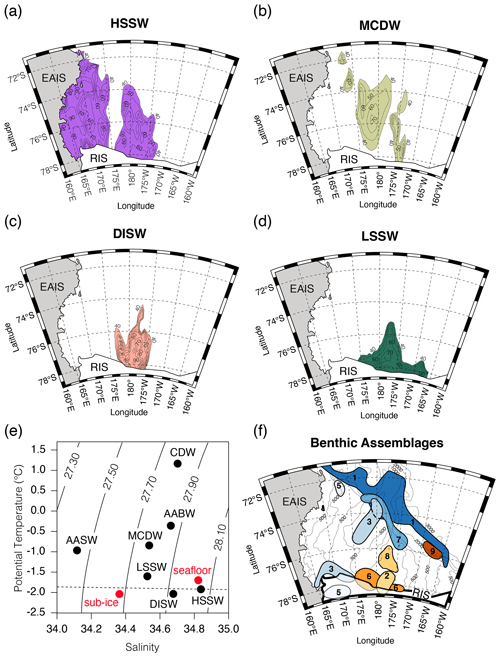
Figure 7Water mass characteristics in relation to prominent benthic foraminiferal assemblages in the Ross Sea. (a–d) Water mass types from Budillon et al. (2003) and (e) Temperature and salinity measurements taken at RISP of the seafloor and sub-ice (red circles) in comparison with water mass types in the Ross Sea (Figure modified from Budillon et al., 2002). (f) Dominant assemblages reported in Osterman and Kellogg (1979), labeled as follow: 1 is Trifarina earlandi, 2 is Hormosina sp., 3 is Globocassidulina subglobosa, 4 is Osangularia umbonifera, 5 is Ehrenbergina glabra, 6 is Miliammina arenacea, 7 is Cibicides lobatulus, 8 is Reophax nodulosus, and 9 is Cyclammina sp. Calcareous assemblages are plotted in cool colors, and agglutinated assemblages are plotted in warm colors. Of note is Assemblage 9 (Cyclammina). Abbreviations are as follows: HSSW stands for High-Salinity Shelf Water, MCDW stands for Modified Circumpolar Deep Water, DISW stands for Deep Ice Shelf Water, and LSSW stands for Low-Salinity Shelf Water.
It is likely that water mass characteristic properties may have a significant influence on assemblages found within sub-ice shelf settings and the proximal grounding line. For example, in Marguerite Bay of the Antarctic Peninsula, agglutinated Cyclammina pusilla and Portatrochammina along with calcareous Globocassidulina biora are found in a sub-ice shelf, grounding zone proximal environment. They also appear in a facies indicative of basal meltwater (Kilfeather et al., 2011; Smith et al., 2019) and perhaps are being influenced by DISW or LSSW, similar to RISP. A shallower CCD (due to extremely cold temperatures) at RISP prevents any calcareous species from precipitating their tests and might explain why G. biora is absent. Only one other study (Osterman and Kellogg, 1979) has reported a rich abundance of Cyclammina (>76 %; Fig. 7f), which is concentrated at ∼1000–2000 m depth on the continental slope at 75°53′ S 165°33′ W in a direct path of LSSW coming into the Whales Deep Basin today. However, it is unlikely the LSSW can reach the depth of the continental slope, and this may be coincidental. Today, Cyclammina spp. (e.g., C. cancellata) is commonly associated with cold bathyal and abyssal settings, and so it is puzzling to see this genus at the shallower depths at RISP. However, other studies in the Atlantic have shown Cyclammina at shallower depths towards higher latitudes (Culver and Buzas, 1980; McNeil, 1988). This suggests that Cyclammina exploits colder temperatures in shelf environments when conditions are optimal. Alternatively, during the Last Glacial Maximum (LGM), the ice sheet was grounded at the edge of the continental shelf. As the ice sheet retreated following the LGM and before isostatic rebound, models show that the shelf was ∼500 m deeper (∼1000 m water depth; Fig. 1c) (Kingslake et al., 2018). This would have provided a potential pathway for Cyclammina on the upper continental slope and directly north of Site J-9 (Osterman and Kellogg, 1979) to follow the retreating grounding zone back to its present location at Site J-9 prior to isostatic rebound of the shelf.
Perhaps the dominance of agglutinated Cyclammina and Cribrostomoides and paucity of calcareous benthics at RISP reflects the presence of salty, corrosive HSSW, which circulated beneath the Ross Ice Shelf and delivered particulate and refractory organic matter from north of the calving front (e.g., Tinto et al., 2019). Such a corrosive water mass may be responsible for the high dominance and low diversity of the Holocene assemblage due in part to carbonate dissolution and the loss of more fragile agglutinated species (Capotondi et al., 2018; Majewski et al., 2016, 2018). Furthermore, Cyclammina has not been reported in Pleistocene or Holocene sediments from the western Ross Sea, which is more saline and slightly warmer. The absence of Cyclammina in other locations in the eastern Ross Sea may be related to a preference towards colder, less saline waters that form only near the grounding zone. Perhaps the waters are too modified by the time they reach the edge of the ice shelf, and we see a different agglutinated assemblage (e.g., Miliammina in the Whales Deep Basin and Hormosina in the Glomar Challenger Trough; Osterman and Kellogg, 1979).
The only other reported occurrences of Cyclammina in the Ross Sea are from DSDP Site 270 in the central Ross Sea. This site was characterized by rapid subsidence associated with rifting during the late Oligocene (Leckie and Webb, 1983, 1986). At the base of the glaciomarine sequence (Unit 2), cold, deep-water agglutinated Cyclammina infiltrated the rapidly subsiding basin, only to be replaced by calcareous benthics, including deep-water Globocassidulina subglobosa, and upper bathyal-neritic taxa (Trifarina fluens, Elphidium magellanicum, Epistominella vitrea, Nonionella spp., Melonis barleeanus, Ammoelphidiella spp., Gyroidina sp.) as the basin filled with glaciomarine sediments during the early Miocene and the shoaling continental shelf gradually prograded northward (Leckie and Webb, 1983, 1986; Bombard et al., 2024).
An additional factor to consider is the relationship between the extent of the ice shelf and food supply. Low foraminiferal numbers in the west Antarctic Peninsula and an increase in the relative abundance of Cyclammina (Kilfeather et al., 2011; Smith et al., 2019) are similar to low foraminiferal counts and dominant C. trullissata at RISP suggesting a response to low organic matter near the grounding zone situated far from the front of the ice shelf. The absence of bioturbation and very low diversity and abundance of marine life at RISP suggest stressed environmental conditions. Amphipods and fish were noted at RISP (Lipps et al., 1979). These likely scavenged upon chemoautotrophic bacteria that fixed nitrates advected to the area (Horrigan, 1981). Cyclammina possibly scavenged on the same bacteria (and possibly on dead amphipods). It may be that water mass, a shallower CCD, and a very low food supply all contributed to the Holocene Cyclammina-dominated assemblage at RISP.
Early observations of the seafloor at RISP (1978–1979), report the temperature near the seafloor was −1.86 °C (0.5 °C above the in situ freezing point) and the salinity was 34.83 ‰ (Jacobs et al., 1979). A mysid shrimp; scavenging, red, gammarid amphipods; an isopod; and two species of fish were present, but no living foraminifera, benthic infauna, or sessile epifauna were observed on the seafloor at Site J-9 (Ronan et al., 1978; Bruchhausen, et al., 1979; Lipps et al., 1979). Several of the large amphipods had no gut contents. No animal traces, current scour, or bioturbation of the sediment were observed. The diversity and abundance of fishes and crustaceans is considerably lower than near the ice shelf edge in McMurdo Sound (Bruchhausen, et al., 1979). In a study of highly oligotrophic microbial ecosystems beneath the Ross Ice Shelf, Martínez-Pérez et al. (2022) concluded that new production of organic carbon is likely chemosynthetically driven by aerobic lithoautotrophic archaea and bacteria that utilize ammonium, nitrate, and sulfur compounds as electron donors. They also found aerobic organo-heterotrophic bacteria that utilized this in situ fixed carbon and current-advected refractory organic matter. Perhaps these autotrophic and heterotrophic archaea and bacteria were the primary food source for the foraminifera and macrofauna at the RISP J-9 sampling site.
Sediments recovered from beneath the Ross Ice Shelf (82°22.5′ S, 168°37.5′ W) at Site J-9 during the austral summers of 1977–1978 and 1978–1979 comprise unstratified, diatom-bearing, sandy-to-silty claystone with dispersed granules and pebbles (diamict) with two distinct units (Webb, 1978, 1979). The upper unit is up to 23 cm thick and is unconsolidated and unstratified with no apparent bioturbation. The lower unit is at least 1 m thick and is a compact, unstratified, diatom-bearing diamict. In addition to dispersed clasts, the lower unit contains common diatomaceous soft sediment clasts (diatomite), dominantly derived from local lower Miocene strata (Harwood et al., 1989). The origin of the sediments, especially the lower unit, may be glaciomarine (Harwood et al., 1989) or till (Sjunneskog and Scherer, 2005). The upper and lower unit are separated by a thin iron-stained contact.
Harwood et al. (1989) demonstrated that the lower unit contains no diatom species that are exclusively diagnostic of the Pliocene or Pleistocene. The well-preserved diatoms in the soft sediment clasts reveal distinct middle early Miocene and early middle Miocene ages, while the matrix contains late Miocene diatoms, indicating its maximum age. In a study of the foraminifera from the RISP cores, Greene (1990) also shows that the age of the lower unit is most likely late Miocene based on the co-occurrence of endemic Miocene planktic species Antarcticella antarctica and polar species Neogloboquadrina pachyderma, as well as endemic Miocene benthic species Ammoelphidiella uniforamina. The most common benthic foraminiferal taxa in the lower unit are Uvigerina sp./Trifarina fluens and Elphidium magellanicum, as well as much lower abundances of Gyroidina sp., Nonionella bradii, N. iridea, Globocassidulina subglobosa, Melonis barleeanus, Globobulimina auriculata, and Epistominella vitrea. These taxa are all diagnostic of the upper Oligocene to middle Miocene of the Ross Sea continental shelf (D'Agostino and Webb, 1980; Leckie and Webb, 1980, 1983, 1986; Steinhauff and Webb, 1987; Galeotti et al., 2000; Strong and Webb, 2000; Webb and Strong, 2006; Patterson and Ishman, 2012; Bombard et al., this volume), suggesting that many or most of these taxa were likely glacially reworked from older deposits, as were the diatom-rich soft sediment clasts. Therefore, foraminifera of the lower unit of the RISP sediments support the diatom biostratigraphy of Harwood et al. (1989), indicating times of open-water conditions in the Ross Embayment south of Site J-9 during the early and middle Miocene, including the Miocene Climatic Optimum (17–14.6 Ma). These ages are correlative to intervals of IODP Sites U1521 and U1522; DSDP Sites 270, 272, and 273; and AND-2A in the central and western Ross Sea.
The upper unit at J-9 is separated from the lower unit by a disconformity based on the unique agglutinated foraminiferal assemblage of the upper unit and the unconsolidated nature of the sediment. The upper unit is most likely post-LGM and probably late Holocene in age (<7 ka), supporting the geochemical findings of Raiswell and Tan (1985). The upper unit was likely deposited in a harsh proximal grounding zone environment as the grounded ice sheet lifted off the seafloor during deglaciation. This ushered in an agglutinated assemblage into the sub-ice cavity consisting of taxa more typical of deeper water environments (e.g., Osterman and Kellogg, 1979). This is a unique relict deglacial, pre-isostatic rebound assemblage dominated by Cyclammina trullissata, C. cf. cancellata, and Cribrostomoides not known from elsewhere in the Ross Sea. This Cyclammina-dominated assemblage may be somewhat analogous with the Cyclammina-dominated assemblage recorded in the basal glaciomarine sediments at DSDP Site 270 and attributed to rapid subsidence of the central Ross Sea during the late Oligocene (Leckie and Webb, 1983, 1986). In the Site 270 case, cold, deep water Cyclammina infiltrated the subsiding Ross Sea from the Southern Ocean but were subsequently replaced by shallower water taxa as the basin filled and the continental shelf prograded northwards. The very rare calcareous specimens in the upper unit, including the planktic N. pachyderma, were likely transported to the site by sub-ice shelf currents, although some of the benthics may have been derived from basal melting of the ice. The sparse Holocene foraminiferal assemblage at Site J-9 may have been specifically adapted to survival in this highly oligotrophic environment due to the availability of microbial resources (Martínez-Pérez et al., 2022). The dominance of agglutinated taxa further suggests that corrosive High-Salinity Shelf Water circulates extensively beneath the Ross Ice Shelf (e.g., Tinto et al., 2019) before cooling and exiting from beneath the ice shelf as Deep Ice Shelf Water (or Low Salinity Shelf Water) (Jacobs et al., 1979; Budillon et al., 2003).
All foraminiferal assemblage data in this paper are reported as tables or in the Supplement. This data can also be accessed via PANGAEA in the future.
The supplement related to this article is available online at: https://doi.org/10.5194/jm-43-187-2024-supplement.
SND performed all sample processing and foraminiferal analysis under the supervision of RML. PNW and RML provided first-hand knowledge on RISP coring operations and core retrieval, and RS and DH provided expert diatom knowledge from prior RISP research. All co-authors were great assets as they have intimate knowledge of RISP and provided great feedback in writing this paper with assistance from RML.
The contact author has declared that none of the authors has any competing interests.
Publisher's note: Copernicus Publications remains neutral with regard to jurisdictional claims made in the text, published maps, institutional affiliations, or any other geographical representation in this paper. While Copernicus Publications makes every effort to include appropriate place names, the final responsibility lies with the authors.
This article is part of the special issue “Advances in Antarctic chronology, paleoenvironment, and paleoclimate using microfossils: Results from recent coring campaigns”. It is not associated with a conference.
A special thanks is given to Val Stanley for providing samples from the Marine and Geology Repository, College of Earth, Ocean, and Atmospheric Sciences, Oregon State University (NSF OPP-1656126). The authors thank the editor Francesca Sangiorgi and reviewers Brian Huber and Giacomo Galli for their helpful feedback. Thanks also to the UMass Micropaleo crew Sam Bombard, Julia Seidenstein, and Erin Kim for helpful discussions and support.
This study was supported by the National Science Foundation grant no. 1947558 “Collaborative Research: Miocene Climate Extremes, A Ross Sea Perspective” to R. Mark Leckie. The original RISP project was supported by a NSF grant (grant no. DP 20657) to Peter-Noel Webb.
This paper was edited by Francesca Sangiorgi and reviewed by Giacomo Galli and Brian Huber.
Anderson, J. B.: Ecology and Distribution of Foraminifera in the Weddell Sea of Antarctica, Micropaleontology, 21, 69–96, https://doi.org/10.2307/1485156, 1975.
Anderson, J. B. and Bartek, L. R.: Cenozoic glacial history of the Ross Sea revealed by intermediate resolution seismic reflection data combined with drill site information, in: The Antarctic Paleoenvironment: A Perspective on Global Change: Part One, edited by: Kennett, J. P. and Warkne, D. A., Antarctic Research Series, 56, 231–263, https://doi.org/10.1029/ar056p0231, 1992.
Anderson, J. B., Conway, H., Bart, P. J., Witus, A. E., Greenwood, S. L., McKay, R. M., Hall, B. L., Ackert, R. P., Licht, K., Jakobsson, M., and Stone, J. O.: Ross Sea paleo-ice sheet drainage and deglacial history during and since the LGM, Quaternary Sci. Rev., 100, 31–54, https://doi.org/10.1016/j.quascirev.2013.08.020, 2014.
Askin, R. A. and Markgraf, V.: Palynomorphs from the Sirius Formation, Dominion Range, Antarctica, Antarct. J. US, 21, 34–35, 1986.
Askin, R. A. and Raine, J. I.: Oligocene and early Miocene terrestrial palynology of the Cape Roberts drillhole CRP-2/2A, Victoria Land Basin, Antarctica, Terra Antarctica, 7, 493–501, 2000.
Barrett, P. J.: Cenozoic climate and sea level history from glacimarine strata off the Victoria Land coast, Cape Roberts Project, Antarctica, in: Glacial Processes and Products, edited by: Hambrey, M. J., Christoffersen, P., Glasser, N. F., and Hubbard, B., International Association of Sedimentologists Special Publication 39, 259–287, 2007.
Barrett, P. J., Elston, D. P., Harwood, D. M., McKelvey, B. C., and Webb, P.-N.: Mid-Cenozoic record of glaciation and sea-level change on the margin of the Victoria Land basin, Antarctica, Geology, 15, 634–637, https://doi.org/10.1130/0091-7613(1987)15<634:mrogas>2.0.co;2, 1987.
Bart, P. J., Coquereau, L., Warny, S., and Majewski, W.: In situ foraminifera in grounding zone diamict: A working hypothesis, Antarct. Sci., 28, 313–321, https://doi.org/10.1017/s0954102016000055, 2016.
Bart, P. J., Krogmeier, B. J., Bart, M. P., and Tulaczyk, S.: The paradox of a long grounding during West Antarctic Ice Sheet retreat in Ross Sea, Sci. Rep.-UK, 7, 1262, https://doi.org/10.1038/s41598-017-01329-8, 2017.
Bombard, S. E., Leckie, R. M., Browne, I. M., Shevenell, A. E., McKay, R. M., Harwood, D. M., and IODP Expedition 374 Scientists: Miocene Climatic Optimum and Middle Miocene Climate Transition: A foraminiferal record from the Central Ross Sea, Antarctica, J. Micropalaeontol., 2024.
Brady, H. T.: Miocene diatom flora from the bottom cores at RISP site J-9, Antarct. J. US, 13, 123–124, 1978.
Brady, H. T.: Diatom biostratigraphy in sediment cores from RISP site J-9, Antarct. J. US, 14, 130, 1979.
Brady, H. T.: Interpretation of sediment cores from the Ross Ice Shelf Site J-9, Antarctica, Nature, 303, 510–511, https://doi.org/10.1038/303510a0, 1983.
Brady, H. T. and Martin, H.: Ross Sea region in the Middle Miocene: A glimpse into the past, Science, 203, 437–438, https://doi.org/10.1126/science.203.4379.437, 1979.
Browning, J. A. and Somerville, D. A.: Access hole drilling through the Ross Ice Shelf, Antarct. J. US, 13, 55, 1978.
Browning, J. A., Bigl, R. A., and Somerville, D. A.: Hot-water drilling and coring at site J-9, Ross Ice Shelf, Antarct. J. US, 14, 60–61, 1979.
Bruchhausen, P. M., Raymond, J. A., Jacobs, S. S., DeVries, A. L., Thorndike, E. M., and DeWitt, H. H.: Fish, Crustaceans, and the Sea Floor Under the Ross Ice Shelf, Science, 203, 449–451, https://doi.org/10.1126/science.203.4379.449, 1979.
Budillon, G., Cordero, S. G., and Salusti, E.: On the dense water spreading off the Ross Sea shelf (Southern Ocean), J. Marine Syst., 35, 207–227, https://doi.org/10.1016/s0924-7963(02)00082-9, 2002.
Budillon, G., Pacciaroni, M., Cozzi, S., Rivaro, P., Catalano, G., Ianni, C., and Cantoni, C.: An optimum multiparameter mixing analysis of the shelf waters in the Ross Sea, Antarct. Sci., 15, 105–118, https://doi.org/10.1017/s095410200300110x, 2003.
Budillon, G., Castagno, P., Aliani, S., Spezie, G., and Padman, L.: Thermohaline variability and Antarctic bottom water formation at the Ross Sea shelf break, Deep-Sea Res. Pt. I, 58, 1002–1018, https://doi.org/10.1016/j.dsr.2011.07.002, 2011.
Capotondi, L., Bergami, C., Giglio, F., Langone, L., and Ravaioli, M.: Benthic foraminifera distribution in the Ross Sea (Antarctica) and its relationship to oceanography, Boll. Soc. Paleontol. I., 57, 187–202, https://doi.org/10.4435/bspi.2018.12, 2018.
Castagno, P., Falco, P., Dinniman, M. S., Spezie, G., and Budillon, G.: Temporal variability of the Circumpolar Deep Water inflow onto the Ross Sea continental shelf, J. Marine Syst., 166, 37–49, https://doi.org/10.1016/j.jmarsys.2016.05.006, 2017.
Chow, J. M. and Bart, P. J.: West Antarctic Ice Sheet grounding events on the Ross Sea outer continental shelf during the middle Miocene, Palaeogeogr. Palaeoecl., 198, 169–186, https://doi.org/10.1016/s0031-0182(03)00400-0, 2003.
Clough, J. W. and Hansen, B. L.: The Ross Ice Shelf Project, Science, 203, 433–434, https://doi.org/10.1126/science.203.4379.433, 1979.
Coccioni, R. and Galeotti, S.: Foraminiferal biostratigraphy and paleoecology of the CIROS-1 Core from McMurdo Sound (Ross Sea, Antarctica), Terra Antarctica, 4, 103–117, 1997.
Coenen, J. J., Scherer, R. P., Warny, S., Castañeda, I. S., Powell, R. D., Tulaczyk, S. M., Puttkammer, R., Hodson, T. O., and Wei, J. H.-C.: Whillans Ice Stream from UpB to WGZ: Siliceous microfossils, palynomorphs, and biomarker evidence of sub-glacial processes and ice stream history, in: AGU Fall Meeting Abstracts, San Francisco, 14–18 December 2015, C11C-0771, 2015.
Conte R., Rebesco, M., De Santis, L., Colleoni, F., Bensi, M., Bergamasco, A., Kovacevic, V., Gales, J., Zgur, F., Accettella, D., De Steur, L., Ursella, L., McKay, R., Kim, S., Lucchi, R. G., and the IODP Expedition 374 Scientists: Bottom current control on sediment deposition between the Iselin Bank and the Hillary Canyon (Antarctica) since the Late Miocene: An integrated seismic-oceanographic approach, Deep-Sea Res. Pt. I, 176, 103606, https://doi.org/10.1016/j.dsr.2021.103606, 2021.
Coxall, H. K., Wilson, P. A., Pälike, H., Lear, C. H., and Backman, J.: Rapid stepwise onset of Antarctic glaciation and deeper calcite compensation in the Pacific Ocean, Nature, 433, 53–57, 2005.
Culver, S. J. and Buzas, M. A.: Distribution of Recent benthic Foraminifera off the North American Atlantic Coast, Smithsonian Contributions to the Marine Sciences, No. 6, Washington, D.C., 512 pp., https://doi.org/10.5479/si.01960768.6.1, 1980.
D'Agostino, A. E.: Foraminiferal biostratigraphy, paleoecology, and systematics of DSDP Site 273, Ross Sea, Antarctica, M.S. thesis, Northern Illinois University, https://huskiecommons.lib.niu.edu/allgraduate-thesesdissertations/3174 (last access: 11 June 2024), 1980.
D'Agostino, A. E. and Webb, P. -N.: Interpretation of mid-Miocene to Recent lithostratigraphy and biostratigraphy at DSDP Site 273, Ross Sea, Antarct. J. US, 15, 118–120, 1980.
Davison, B. J., Hogg, A. E., Gourmelen, N., Jakob, L., Wuite, J., Nagler, T., Greene, C. A., Andreasen, J., and Engdahl, M. E.: Annual mass budget of Antarctic ice shelves from 1997 to 2021, Sci. Adv., 9, eadi0186, https://doi.org/10.1126/sciadv.adi0186, 2023.
De Angelis, H. and Skvarca, P.: Glacier surge after ice shelf collapse, Science, 299, 1560–1562, https://doi.org/10.1126/science.1077987, 2003.
DeConto, R. M. and Pollard, D.: Rapid Cenozoic glaciation of Antarctica induced by declining atmospheric CO2, Nature, 42, 245–249, 2003.
DeConto, R. M. and Pollard, D.: Contribution of Antarctica to past and future sea-level rise, Nature, 531, 591–597, https://doi.org/10.1038/nature17145, 2016.
DeConto, R. M., Pollard, D., and Kowalewski, D.: Modeling Antarctic ice sheet and climate variations during Marine Isotope Stage 31, Global Planet. Change, 88, 45–52, https://doi.org/10.1016/j.gloplacha.2012.03.003, 2012.
De Santis, L., Anderson, J. B., Brancolini, G., and Zayatz, I.: Seismic record of late Oligocene through Miocene glaciation on the central and eastern continental shelf of the Ross Sea, in: Geology and Seismic Stratigraphy of the Antarctic Margin, edited by: Cooper, A. K., Barker, P. F., and Brancolini, G., Antar. Res. S., 68, 235–260, https://doi.org/10.1029/ar068p0235, 1995.
Duncan, B., McKay, R., Levy, R., Naish, T., Prebble, J. G., Sangiorgi, F., Krishnan, S., Hoem, F., Clowes, C., Jones, T. D., Gasson, E., Kraus, C., Kulhanek, D. K., Meyers, S. R., Moossen, H., Warren, C., Willmott, V., Ventura, G. T., and Bendle, J.: Climatic and tectonic drivers of late Oligocene Antarctic ice volume, Nat. Geosci., 15, 819–825, https://doi.org/10.1038/s41561-022-01025-x, 2022.
Fielding, C. R. and Thomson, M. R. A.: Studies from Cape Roberts Project Initial Report on CRP-2/2A, Ross Sea, Antarctica, Terra Antarctica, 6, 1–173, 1999.
Fielding, C. R., Naish, T. R., Woolfe, K. J., and Lavelle, M. A.: Facies analysis and sequence stratigraphy of CRP-2/2A, Victoria Land Basin, Terra Antarctica, 7, 323–338, 2000.
Fillon, R. H.: Late Cenozoic paleo-oceanography of the Ross Sea, Antarctica, Geol. Soc. Am. Bull., 86, 839–845, https://doi.org/10.1130/0016-7606(1975)86<839:lcpotr>2.0.co;2, 1975.
Galeotti, S. and Coccioni, R.: Foraminiferal analysis of the Miocene CRP-1 Core (Ross, Sea, Antarctica), Terra Antarctica, 5, 521–526, 1998.
Galeotti, S., Cita, M. B., and Coccioni, R.: Foraminiferal biostratigraphy and palaeoecology from two intervals of the CRP2/2A Drilhole, Victoria Land Basin, Antarctica, Terra Antarctica, 7, 473–478, 2000.
Galeotti, S., DeConto, R., Naish, T., Stocchi, P., Florindo, F., Pagani, M., Barrett, P., Bohaty, S. M., Lanci, L., Pollard, D., Sandroni, S., Talarico, F. M., and Zachos, J. C.: Antarctic Ice Sheet variability across the Eocene-Oligocene boundary climate transition, Science, 352, 76–80, https://doi.org/10.1126/science.aab0669, 2016.
Gasson, E., DeConto, R. M., Pollard, D., and Levy, R. H.: Dynamic Antarctic ice sheet during the early to mid-Miocene, P. Natl. Acad. Sci. USA, 113, 3459–3464, https://doi.org/10.1073/pnas.1516130113, 2016.
Gilmour, A. E.: Ross Ice Shelf Sea Temperatures, Science, 203, 438–439, https://doi.org/10.1126/science.203.4379.438, 1979.
Greene, D. G.: Foraminiferal Biostratigraphy and Paleoecology of the Ross Ice Shelf Project Site J9, unpublished M.S. thesis, Ohio State University, 1990.
Greenwood, S. L., Simkins, L. M., Halberstadt, A. R. W., Prothro, L. O., and Anderson, J. B.: Holocene reconfiguration and readvance of the East Antarctic Ice Sheet, Nat. Commun., 9, 3176, https://doi.org/10.1038/s41467-018-05625-3, 2018.
Halberstadt, A. R. W., Simkins, L. M., Greenwood, S. L., and Anderson, J. B.: Past ice-sheet behaviour: retreat scenarios and changing controls in the Ross Sea, Antarctica, The Cryosphere, 10, 1003–1020, https://doi.org/10.5194/tc-10-1003-2016, 2016.
Halberstadt, A. R. W., Chorley, H., Levy, R. H., Naish, T., DeConto, R. M., Gasson, E., and Kowalewski, D. E.: CO2 and tectonic controls on Antarctic climate and ice-sheet evolution in the mid-Miocene, Earth Planet. Sc. Lett., 564, 116908, https://doi.org/10.1016/j.epsl.2021.116908, 2021.
Halberstadt, A. R. W., Kowalewski, D. E., and DeConto, R. M.: Reconciling persistent sub-zero temperatures in the McMurdo Dry Valleys, Antarctica, with Neogene dynamic marine ice-sheet fluctuations, Geology, 50, 557–561, https://doi.org/10.1130/g49664.1, 2022.
Hartman, J. D., Sangiorgi, F., Salabarnada, A., Peterse, F., Houben, A. J. P., Schouten, S., Brinkhuis, H., Escutia, C., and Bijl, P. K.: Paleoceanography and ice sheet variability offshore Wilkes Land, Antarctica – Part 3: Insights from Oligocene–Miocene TEX86-based sea surface temperature reconstructions, Clim. Past, 14, 1275–1297, https://doi.org/10.5194/cp-14-1275-2018, 2018.
Hammer, Ø., Harper, D. A. T., and Ryan, P. D.: PAST: Paleontological statistics software package for education and data analysis, Palaeontol. Eelectron., 4, 1–9, 2001.
Harwood, D. M. and Scherer, R. P.: Diatom biostratigraphy and paleoenvironmental significance of reworked Miocene diatomaceous clasts in sediments from RISP site J-9, Antarct. J. US, 23, 31–34, 1988.
Harwood, D. M., Scherer, R. P., and Webb, P.-N.: Multiple Miocene marine productivity events in West Antarctica as recorded in upper Miocene sediments beneath the Ross Ice Shelf (Site J-9), Mar. Micropaleontol., 15, 91–115, https://doi.org/10.1016/0377-8398(89)90006-6, 1989.
Hattermann, T., Nøst, O. A., Lilly, J. M., and Smedsrud, L. H.: Two years of oceanic observations below the Fimbul Ice Shelf, Antarctica, Geophys. Res. Lett., 39, L12605, https://doi.org/10.1029/2012gl051012, 2012.
Hayes, D. E. and Frakes, L. A.: General synthesis, Deep Sea Drilling Project Leg 28, in: Initial Reports of the Deep Sea Drilling Project, 28, edited by: Hayes, D. E., Frakes, L. A., Barrett, P. J., Burns, D. A., Chen, P.-H., Ford, A. B., Kaneps, A. G., Kemp, E. M., McCollum, D. W., Piper, D. J. W., Wall, R. E., Webb, P. N., Kemp, E. M., McCollum, D. W., Piper, D. J. W., Wall, R. E., and Webb, P. N., U.S. Government Printing Office, Washington, D.C., 919–942, https://doi.org/10.2973/dsdp.proc.28.136.1975, 1975.
Hayward, B. W., Grenfell, H. R., Reid, C. M., and Hayward, K. A.: Recent New Zealand Shallow-Water Benthic Foraminifera: Taxonomy, Ecologic Distribution, Biogeography, and use in Paleoenvironmental Assessment, New Zealand Geological Survey Paleontological Bulletin, 75, 258 pp., 1999.
Horrigan, S. G.: Primary production under the Ross Ice Shelf, Antarctica, Limnol. Oceanogr., 26, 378–382, https://doi.org/10.4319/lo.1981.26.2.0378, 1981.
Holland, P. R., Bracegirdle, T. J., Dutrieux, P., Jenkins, A., and Steig, E. J.: West Antarctic ice loss influenced by internal climate variability and anthropogenic forcing, Nat. Geosci., 12, 718–724, https://doi.org/10.1038/s41561-019-0420-9, 2019.
Houtz, R. and Meijer, R.: Structure of the Ross Sea Shelf from profiler data, J. Geophys. Res., 75, 6592–6597, https://doi.org/10.1029/jb075i032p06592, 1970.
Houtz, R. E. and Davey, F. J.: Seismic profiler and sonobuoy measurements in Ross Sea, Antarctica, J. Geophys. Res., 78, 3448–3468, https://doi.org/10.1029/jb078i017p03448, 1973.
Ishman, S. E. and Webb, P.-N.: Late Neogene foraminiferal record and geological history inferred from Dry Valley Drilling Cores 10 and 11, Taylor Valley, Antarctica, Antarct. J. US, 21, 13–15, 1986.
Ishman, S. E. and Webb, P.-N.: Late Neogene benthic foraminifera from the Victoria land basin margin, Antarctica- Application to glacio-eustatic and tectonic events, Revue de Paléobiologie, Volume speìcial 2, Benthos, 86, 523–551, 1988.
Jacobs, S., Bruchhausen, P., and Ardai, J.: Physical oceanography of the Ross Sea, Antarct. J. US, 13, 83–85, 1978.
Jacobs, S. S., Gordon, A. L., and Ardai Jr., J. L. A.: Circulation and Melting Beneath the Ross Ice Shelf, Science, 203, 439–443, https://doi.org/10.1126/science.203.4379.439, 1979.
Jacobs, S. S., Helmer, H. H., Doake, C. S. M., Jenkins, A., and Frolich, R. M.: Melting of ice shelves and the mass balance of Antarctica, J. Glaciol., 38, 375–387, https://doi.org/10.3189/s0022143000002252, 1992.
Jiang, X. and Harwood, D. M.: A glimpse of early Miocene Antarctic forests: Palynomorphs from RISP diatomite, Antarct. J. US, 27, 3–6, 1993.
Johnson, J. S., Venturelli, R. A., Balco, G., Allen, C. S., Braddock, S., Campbell, S., Goehring, B. M., Hall, B. L., Neff, P. D., Nichols, K. A., Rood, D. H., Thomas, E. R., and Woodward, J.: Review article: Existing and potential evidence for Holocene grounding line retreat and readvance in Antarctica, The Cryosphere, 16, 1543–1562, https://doi.org/10.5194/tc-16-1543-2022, 2022.
Kellogg, D. E. and Kellogg, T. B.: Revised age for RISP sediments and implications for the glacial history of Antarctica, Antarct. J. US, 16, 61–63, 1980.
Kellogg, T. B. and Kellogg, D. E.: Pleistocene sediments beneath the Ross Ice Shelf, Nature, 293, 130–133, https://doi.org/10.1038/293130a0, 1981.
Kellogg, T. B. and Kellogg, D. E.: Interpretation of sediment cores from the Ross Ice Shelf Site J-9, Antarctica: Reply by Thomas B. Kellogg & Davida E. Kellogg, Nature, 303, 511–513, https://doi.org/10.1038/303511a0, 1983.
Kellogg, D. E. and Kellogg, T. B.: Diatom biostratigraphy of sediment cores from beneath the Ross Ice Shelf, Micropaleontology, 32, 74–94, https://doi.org/10.2307/1485703, 1986.
Kemp, E. M. and Barrett, P. J.: Antarctic glaciation and early Tertiary vegetation, Nature, 258, 507–508, https://doi.org/10.1038/258507a0, 1975.
Kennett, J. P.: Fauna of the Ross Sea; Ecology and Distribution of Foraminifera; Ecology and Distribution of Foraminifera, Part 6, New Zealand Department of Scientific and Industrial Research Bulletin, 186, 48 pp., 1968.
Kennett, J. P.: Cenozoic evolution of Antarctic glaciation, the circum-Antarctic Ocean, and their impact on global paleoceanography, J. Geophys. Res., 82, 3843–3860, https://doi.org/10.1029/jc082i027p03843, 1977.
Kennett, J. P.: The development of planktonic biogeography in the Southern Ocean during the Cenozoic, Mar. Micropaleontol., 3, 301–345, 1978.
Kilfeather, A. A., Cofaigh, C. Ó., Lloyd, J. M., Dowdeswell, J. A., Xu, S., and Moreton, S. G.: Ice-stream retreat and ice-shelf history in Marguerite Trough, Antarctic Peninsula: Sedimentological and foraminiferal signatures, Geol. Soc. Am. Bull., 123, 997–1015, https://doi.org/10.1130/b30282.1, 2011.
Kingslake, J., Scherer, R. P., Albrecht, T., Coenen, J., Powell, R. D., Reese, R., Stansell, N. D., Tulaczyk, S., Wearing, M. G., and Whitehouse, P. L.: Extensive retreat and re-advance of the West Antarctic Ice Sheet during the Holocene, Nature, 558, 430–434, https://doi.org/10.1038/s41586-018-0208-x, 2018.
Koci, B. R.: Hot water drilling in Antarctic firn, and freezing rates in water-filled boreholes, CRREL Special Report 84-34, 101–103, 1982.
Kucera, M. and Schönfeld, J.: The origin of modern oceanic foraminiferal faunas and Neogene climate change, in: Deep-Time Perspectives on Climate Change: Marrying the Signal from Computer Models and Biological Proxies, edited by: Williams, M., Haywood, A. M., Gregory, F. J., and Schmidt, D. N., The Micropalaeontological Society, Special Publications, 2, 409–425, https://doi.org/10.1144/tms002, 2007.
Kulhanek, D. K., Levy, R. H., Clowes, C. D., Prebble, J. G., Rodelli, D., Jovane, L., Morgans, H. E. G., Kraus, C., Zwingmann, H., Griffith, E. M., Scher, H. D., McKay, R. M., and Naish, T. R.: Revised chronostratigraphy of DSDP Site 270 and late Oligocene to early Miocene paleoecology of the Ross Sea sector of Antarctica, Global Planet. Change, 178, 46–64, https://doi.org/10.1016/j.gloplacha.2019.04.002, 2019.
Leckie, R. M. and Olson, H. C.: Foraminifera as proxies for sea-level change on siliciclastic margins, in: Micropaleontologic Proxies for Sea-Level Change and Stratigraphic Discontinuities, edited by: Olson, H. C. and Leckie, R. M., SEPM Society for Sedimentary Geology Special Publication No. 75, 5–19, https://doi.org/10.2110/pec.03.75.0005, 2003.
Leckie, R. M. and Webb, P.-N.: Foraminifera of DSDP Site 270 as indicators of the evolving Ross Sea in the late Oligocene/early Miocene, Antarct. J. US, 15, 117–118, 1980.
Leckie, R. M. and Webb, P.-N.: Late Oligocene–early Miocene glacial record of the Ross Sea, Antarctica: Evidence from DSDP Site 270, Geology, 11, 578–582, https://doi.org/10.1130/0091-7613(1983)11<578:lomgro>2.0.co;2, 1983.
Leckie, R. M. and Webb, P.-N.: Candeina antarctica, n. sp. and the phylogenetic history and distribution of Candeina spp. in the Paleogene-early Neogene of the Southern Ocean, J. Foramin. Res., 15, 65–78, https://doi.org/10.2113/gsjfr.15.2.65, 1985.
Leckie, R. M. and Webb, P.-N.: Late Paleogene and Early Neogene foraminifers of Deep Sea Drilling Project Site 270, Ross, Antarctica, in: Initial Reports of the Deep Sea Drilling Project, 90, edited by: Kennett, J. P., von der Borch, C. C., Baker, P. A., U. S. Government Printing Office, Washington, D.C., 1093–1142, https://doi.org/10.2973/dsdp.proc.90.124.1986, 1986.
Levy, R., Harwood, D., Florindo, F., Sangiorgi, F., Tripati, R., von Eynatten, H., Gasson, E., Kuhn, G., Tripati, A., DeConto, R., Fielding, C., Field, B., Golledge, N., McKay, R., Naish, T., Olney, M., Pollard, D., Schouten, S., Talarico, F., Warny, S., Willmott, V., Acton, G., Panter, K., Paulsen, T., Taviani, M., and SMS Science Team: Antarctic ice sheet sensitivity to atmospheric CO2 variations in the early to mid-Miocene, P. Natl. Acad. Sci. USA, 113, 3453–3458, https://doi.org/10.1073/pnas.1516030113, 2016.
Levy, R. H., Meyers, S. R., Naish, T. R., Golledge, N. R., McKay, R. M., Crampton, J. S., DeConto, R. M., De Santis, L., Florindo, F., Gasson, E. G. W., Harwood, D. M., Luyendyk, B. P., Powell, R. D., Clowes, C., and Kulhanek, D. K.: Antarctic ice-sheet sensitivity to obliquity forcing enhanced through ocean connections, Nat. Geosci., 12, 132–137, https://doi.org/10.1038/s41561-018-0284-4, 2019.
Ling, H. Y. and White, R. J.: Silicoflagellate Mesocena pappii identified in RISP site J-9 core sediments, Antarct. J. US, 145, 126–127, 1979.
Lipps, J. H., Ronan Jr., T. E., and Delaca, T. E.: Life below the Ross Ice Shelf, Antarctica, Science, 203, 447–449, https://doi.org/10.1126/science.203.4379.447, 1979.
Lowry, D. P., Golledge, N. R., Bertler, N. A. N., Jones, R. S., and McKay, R.: Deglacial grounding-line retreat in the Ross Embayment, Antarctica, controlled by ocean and atmosphere forcing, Sci. Adv., 5, eaav8754, https://doi.org/10.1126/sciadv.aav8754, 2019.
Majewski, W.: Benthic foraminifera from Pine Island and Ferrero bays, Amundsen Sea, Pol. Polar Res., 34, 169–200, https://doi.org/10.2478/popore-2013-0012, 2013.
Majewski, W., Wellner, J. S., and Anderson, J. B.: Environmental connotations of benthic foraminiferal assemblages from coastal West Antarctica, Mar. Micropaleontol., 124, 1–15, https://doi.org/10.1016/j.marmicro.2016.01.002, 2016.
Majewski, W., Bart, P. J., and McGlannan, A. J.: Foraminiferal assemblages from ice-proximal paleo-settings in the Whales Deep Basin, eastern Ross Sea, Antarctica, Palaeogeogr. Palaeocl., 493, 64–81, https://doi.org/10.1016/j.palaeo.2017.12.041, 2018.
Majewski, W., Prothro, L. O., Simkins, L. M., Demianiuk, E. J., and Anderson, J. B.: Foraminiferal patterns in deglacial sediment in the western Ross Sea, Antarctica: Life near grounding lines, Paleoceanogr. Paleocl., 35, e2019PA003716, https://doi.org/10.1029/2019pa003716, 2020.
Marschalek, J. W., Zurli, L., Talarico, F., van de Flierdt, T., Vermeesch, P., Carter, A., Beny, F., Bout-Roumazeilles, V., Sangiorgi, F., Hemming, S. R., Pérez, L. F., Colleoni, F., Prebble, J. G., van Peer, T. E., Perotti, M., Shevenell, A. E., Browne, I., Kulhanek, D. K., Levy, R., Harwood, D., Sullivan, N. B., Meyers, S. R., Griffith, E. M., Hillenbrand, C.-D., Gasson, E., Siegert, M. J., Keisling, B., Licht, K. J., Kuhn, G., Dodd, J. P., Boshuis, C., De Santis, L., McKay, R. M., and IODP Expedition 374: A large West Antarctic Ice Sheet explains early Neogene sea-level amplitude, Nature, 600, 450–455, https://doi.org/10.1038/s41586-021-04148-0, 2021.
Martínez-Pérez, C., Greening, C., Bay, S. K., Lappan, R. J., Zhao, Z., Corte, D. D., Hulbe, C., Ohneiser, C., Stevens, C., Thomson, B., Stepanauskas, R., González, J. M., Logares, R., Herndl, G. J., Morales, S. E., and Baltar, F.: Phylogenetically and functionally diverse microorganisms reside under the Ross Ice Shelf, Nat. Commun., 13, 117, https://doi.org/10.1038/s41467-021-27769-5, 2022.
McCollum, D. W.: Diatom stratigraphy of the Southern Ocean, in: Initial Reports of the Deep Sea Drilling Project, 28, edited by: Hayes, D. E., Frakes, L. A., Barrett, P. J., Burns, D. A., Chen, P.-H., Ford, A. B., Kaneps, A. G., Kemp, E. M., McCollum, D. W., Piper, D. J. W., Wall, R. E., and Webb, P. N., U.S. Government Printing Office, Washington, D.C., 515–571, https://doi.org/10.2973/dsdp.proc.28.112.1975, 1975.
McGlannan, A. J., Bart, P. J., Chow, J. M., and DeCesare, M.: On the influence of post-LGM ice shelf loss and grounding zone sedimentation on West Antarctic ice sheet stability, Mar. Geol., 392, 151–169, https://doi.org/10.1016/j.margeo.2017.08.005, 2017.
McKay, R. M., Santis, L. D., Kulhanek, D. K., Ash, J. L., Beny, F., Browne, I. M., Cortese, G., de Sousa, I. M. C., Dodd, J. P., Esper, O. M., Gales, J. A., Harwood, D. M., Ishino, S., Keisling, B. A., Kim, S., Laberg, J. S., Leckie, R. M., Müller, J., Patterson, M. O., Romans, B. W., Romero, O. E., Sangiorgi, F., Seki, O., Shevenell, A. E., Singh, S. M., Sugisaki, S. T., van de Flierdt, T., van Peer, T. E., Xiao, W., and Xiong, Z.: Expedition 374 summary, in: Ross Sea West Antarctic Ice Sheet History, edited by: McKay, R. M., De Santis, L., Kulhanek, D. K., and Expedition 374 Scientists, Proceedings of the International Ocean Discovery Program, 374, College Station, TX (International Ocean Discovery Program), https://doi.org/10.14379/iodp.proc.374.101.2019, 2019.
McNeil, D. H.: Cyclammina cyclops, n. sp., in the Eocene Richards Formation, Beaufort Sea area of Arctic Canada, J. Foramin. Res., 18, 114–123, https://doi.org/10.2113/gsjfr.18.2.114, 1988.
Melis, R. and Salvi, G.: Late Quaternary foraminiferal assemblages from western Ross Sea (Antarctica) in relation to the main glacial and marine lithofacies, Mar. Micropaleontol., 70, 39–53, https://doi.org/10.1016/j.marmicro.2008.10.003, 2009.
Mildenhall, D. C.: Terrestrial palynology, in: Antarctic Cenozoic History from the CIROS-1 Drillhole, McMurdo Sound, edited by: Barrett, P. J., DSIR Bulletin, 245, 119–127, 1989.
Murray, J. W.: Ecology and Palaeoecology of Benthic Foraminifera, Longman Scientific & Technical, London, 397 pp., https://doi.org/10.4324/9781315846101, 1991.
Naish, T., Powell, R., Levy, R., Wilson, G., Scherer, R., Talarico, F., Krissek, L., Niessen, F., Pompilio, M., Wilson, T., Carter, L., DeConto, R., Huybers, P., McKay, R., Pollard, D., Ross, J., Winter, D., Barrett, P. J., Browne, G., Cody, R., Cowan, E., Crampton, J., Dunbar, G., Dunbar, N., Florindo, F., Gebhardt, C., Graham, I., Hannah, M., Hansaraj, D., Harwood, D., Helling, D., Henrys, S., Hinnov, L., Kuhn, G., Kyle, P., Läufer, A., Maffioli, P., Magens, D., Mandernack, K., McIntosh, W., Millan, C., Morin, R., Ohneiser, C., Paulsen, T., Persico, D., Raine, I., Reed, J., Riesselman, C., Sagnotti, L., Schmitt, D., Sjunneskog, C., Strong, P., Taviani, M., Vogel, S., Wilch, T., and Williams, T.: Obliquity-paced Pliocene West Antarctic ice sheet oscillations, Nature, 458, 322–328, https://doi.org/10.1038/nature07867, 2009.
Neuhaus, S. U., Tulaczyk, S. M., Stansell, N. D., Coenen, J. J., Scherer, R. P., Mikucki, J. A., and Powell, R. D.: Did Holocene climate changes drive West Antarctic grounding line retreat and readvance?, The Cryosphere, 15, 4655–4673, https://doi.org/10.5194/tc-15-4655-2021, 2021.
Olivetti, V., Balestrieri, M. L., Chew, D., Zurli, L., Zattin, M., Pace, D., Drakou, F., Cornamusini, G., and Perotti, M.: Ice volume variations and provenance trends in the Oligocene-early Miocene glaciomarine sediments of the Central Ross Sea, Antarctica (DSDP Site 270), Global Planet. Change, 221, 104042, https://doi.org/10.1016/j.gloplacha.2023.104042, 2023.
Orsi, A. H. and Wiederwohl, C. L.: A recount of Ross Sea waters, Deep-Sea Res. Pt. II, 56, 778–795, https://doi.org/10.1016/j.dsr2.2008.10.033, 2009.
Osterman, L. E. and Kellogg, T. B.: Recent benthic foraminiferal distributions from the Ross Sea, Antarctica: Relation to ecologic and oceanographic conditions, J. Foramin. Res., 9, 250–269, https://doi.org/10.2113/gsjfr.9.3.250, 1979.
Patterson, M. O. and Ishman, S. E.: Neogene benthic foraminiferal assemblages and paleoenvironmental record for McMurdo Sound, Antarctica, Geosphere, 8, 1331–1341, https://doi.org/10.1130/ges00771.1, 2012.
Patterson, M. O., Levy, R. H., Kulhanek, D. K., van de Flierdt, T., Horgan, H., Dunbar, G. B., Naish, T. R., Ash, J., Pyne, A., Mandeno, D., Winberry, P., Harwood, D. M., Florindo, F., Jimenez-Espejo, F. J., Läufer, A., Yoo, K.-C., Seki, O., Stocchi, P., Klages, J. P., Lee, J. I., Colleoni, F., Suganuma, Y., Gasson, E., Ohneiser, C., Flores, J.-A., Try, D., Kirkman, R., Koch, D., and the SWAIS 2C Science Team: Sensitivity of the West Antarctic Ice Sheet to +2 °C (SWAIS 2C), Sci. Dril., 30, 101–112, https://doi.org/10.5194/sd-30-101-2022, 2022.
Paxman, G. J. G.: Antarctic palaeotopography, Geological Society, London, Memoirs, 56, 231–251, https://doi.org/10.1144/m56-2020-7, 2023.
Pollard, D. and DeConto, R. M.: Modelling West Antarctic ice sheet growth and collapse through the past five million years, Nature, 458, 329–332, https://doi.org/10.1038/nature07809, 2009.
Prebble, J. G., Raine, J. I., Barrett, P. J., and Hannah, M. J.: Vegetation and climate from two Oligocene glacioeustatic sedimentary cycles (31 and 24 Ma) cored by the Cape Roberts Project, Victoria Land Basin, Antarctica, Palaeogeogr. Palaeocl., 231, 41–57, https://doi.org/10.1016/j.palaeo.2005.07.025, 2006.
Pritchard, H. D., Ligtenberg, S. R. M., Fricker, H. A., Vaughan, D. G., Broeke, M. R. van den, and Padman, L.: Antarctic ice-sheet loss driven by basal melting of ice shelves, Nature, 484, 502–505, https://doi.org/10.1038/nature10968, 2012.
Prothro, L. O., Simkins, L. M., Majewski, W., and Anderson, J. B.: Glacial retreat patterns and processes determined from integrated sedimentology and geomorphology records, Mar. Geol., 395, 104–119, https://doi.org/10.1016/j.margeo.2017.09.012, 2018.
Raine, J. I. and Askin, R. A.: Terrestrial palynology: Age and paleoenvironmental results from CRP-3, Victoria Land Basin, Antarctica, Terra Antarctica, 8, 389–400, 2001.
Raiswell, R. and Tan, M. M.: Diagenesis of sediments beneath the Ross Ice Shelf and their sedimentary history, Nature, 315, 483–485, https://doi.org/10.1038/315483a0, 1985.
Rand, J. H.: Ross Ice Shelf Project drilling, October–December 1976, Antarct. J. US, 12, 150–152, 1977.
Ridha, D., Boomer, I., and Edgar, K. M.: Latest Oligocene to earliest Pliocene deep-sea benthic foraminifera from Ocean Drilling Program (ODP) Sites 752, 1168 and 1139, southern Indian Ocean, J. Micropalaeontol., 38, 189–229, https://doi.org/10.5194/jm-38-189-2019, 2019.
Ronan Jr., T. E., Lipps, J. H., and DeLaca, T. E.: Sediments and life under the Ross Ice Shelf (J-9), Antarctica, Antarct. J. US, 13, 141–142, 1978.
Sangiorgi, F., Bijl, P. K., Passchier, S., Salzmann, U., Schouten, S., McKay, R., Cody, R. D., Pross, J., Flierdt, T. van de, Bohaty, S. M., Levy, R., Williams, T., Escutia, C., and Brinkhuis, H.: Southern Ocean warming and Wilkes Land ice sheet retreat during the mid-Miocene, Nat. Commun., 9, 317, https://doi.org/10.1038/s41467-017-02609-7, 2018.
Savage, M. L. and Ciesielski, P. F.: A revised history of glacial sedimentation in the Ross Sea region, in: Antarctic Earth Science, edited by: Oliver, R. L., James, P. R., and Jago, J. B., Cambridge University Press, Cambridge, 555–559, 1983.
Scherer, R. P.: Quaternary and Tertiary microfossils from beneath Ice Stream B: Evidence for a dynamic West Antarctic Ice Sheet history, Global Planet. Change, 4, 395–412, https://doi.org/10.1016/0921-8181(91)90005-h, 1991.
Scherer, R. P., Harwood, D. M., Ishman, S. E., and Webb, P.-N.: Micropaleontological analysis of sediments from the Crary Ice Rise, Ross Ice Shelf, Antarct. J. US, 23, 34–36, 1988.
Scherer, R. P., Aldahan, A., Tulaczyk, S., Possnert, G., Engelhardt, H., and Kamb, B.: Pleistocene Collapse of the West Antarctic Ice Sheet, Science, 281, 82–85, https://doi.org/10.1126/science.281.5373.82, 1998.
Scherer, R. P., Sjunneskog, C. M., Iverson, N. R., and Hooyer, T. S.: Assessing subglacial processes from diatom fragmentation patterns, Geology, 32, 557–560, https://doi.org/10.1130/g20423.1, 2004.
Schrader, H.-J.: Cenozoic planktonic diatom biostratigraphy of the southern Pacific Ocean, in: Initial Reports of the Deep Sea Drilling Project, 35, edited by: Hollister, C. D., Craddock, C., Bogdanov, Y. A., Edgar, N. T., Gieskes, J. M., Haq, B. U., Lawrence, J. R., Rögl, F., Schrader, H.-J., Tucholke, B. E., Vennum, W. R., Weaver, F. M., and Zhivago, V. N., U.S. Government Printing Office, Washington, D.C., 605–671, https://doi.org/10.2973/dsdp.proc.35.136.1976, 1976.
Seidenstein, J., Leckie, R. M., McKay, R., De Santis, L., Harwood, D., and Exp 374 Scientists: Pliocene-Pleistocene warm water incursions and water mass changes on the Ross Sea continental shelf (Antarctica) based on foraminifera, IODP Exp 374, J. Micropalaeontol., 2024.
Semensatto, D. L. and Dias-Brito, D.: Alternative saline solutions to float foraminiferal tests, J. Foramin. Res., 37, 265–269, https://doi.org/10.2113/gsjfr.37.3.265, 2007.
Sjunneskog, C. and Scherer, R. P.: Mixed diatom assemblages in glacigenic sediment from the central Ross Sea, Antarctica, Palaeogeogr. Palaeocl., 218, 287–300, https://doi.org/10.1016/j.palaeo.2004.12.019, 2005.
Smith, J. A., Graham, A. G. C., Post, A. L., Hillenbrand, C.-D., Bart, P. J., and Powell, R. D.: The marine geological imprint of Antarctic ice shelves, Nat. Commun., 10, 5635, https://doi.org/10.1038/s41467-019-13496-5, 2019.
Smith, W., Sedwick, P., Arrigo, K., Ainley, D., and Orsi, A.: The Ross Sea in a sea of change, Oceanography, 25, 90–103, https://doi.org/10.5670/oceanog.2012.80, 2012.
Snyder, S. W. and Huber, B. T.: Preparation techniques for use of foraminifera in the classroom, Paleontological Soc. Pap., 2, 231–236, https://doi.org/10.1017/s1089332600003302, 1996.
Steinhauff, D. M.: Paleoecologic and biostratigraphic analysis of Miocene Foraminifera (Protozoa) from glacial-marine sediments, Ross Sea, Antarctica, Unpublished MS Thesis, Ohio State University, 1985.
Steinhauff, D. M. and Webb, P.-N.: Miocene foraminifera from DSDP Site 272 Ross Sea, Antarct. J. US, 22, 125–126, 1987.
Stern, A. A., Dinniman, M. S., Zagorodnov, V., Tyler, S. W., and Holland, D. M.: Intrusion of warm surface water beneath the McMurdo Ice Shelf, Antarctica, J. Geophys. Res.-Oceans, 118, 7036–7048, https://doi.org/10.1002/2013jc008842, 2013.
Stewart, C. L., Christoffersen, P., Nicholls, K. W., Williams, M. J. M., and Dowdeswell, J. A.: Basal melting of Ross Ice Shelf from solar heat absorption in an ice-front polynya, Nat. Geosci., 12, 435–440, https://doi.org/10.1038/s41561-019-0356-0, 2019.
Stickley, C. E., Brinkhuis, H., Schellenberg, S. A., Sluijs, A., Röhl, U., Fuller, M., Grauert, M., Huber, M., Warnaar, J., and Williams, G. L.: Timing and nature of the deepening of the Tasmanian Gateway, Paleoceanography, 19, PA4027, https://doi.org/10.1029/2004PA001022, 2004.
Strong, C. P. and Webb, P.-N.: Lower Miocene foraminifera from CRP-1 drillhole, Terra Antarctica, 5, 515–520, 1998.
Strong, C. P. and Webb, P.-N.: Oligocene and Miocene foraminifera from CRP-2:2A, Victoria Land Basin, Antarctica, Terra Antarctica, 7, 461–472, 2000.
Strong, C. P. and Webb, P.-N.: Lower Oligocene foraminiferal fauna from CRP-3 Drillhole, Victoria Land Basin, Antarctica, Terra Antarctica, 8, 347–358, 2001.
Taviani, M., Hannah, M., Harwood, D. M., Ishman, S. E., Johnson, K., Olney, M., Riesselman, C., Tuzzi, E., Askin, R., Beu, A. G., Blair, S., Cantarelli, V., Ceregato, A., Corrado, S., Mohr, B., Nielsen, S. H. H., Persico, D., Petrushak, S., Raine, J. I., Warny, S., and ANDRILL-SMS Science Team: Palaeontological characterisation and analysis of the AND-2A Core, ANDRILL Southern McMurdo Sound Project, Antarctica, Terra Antarctica, 15, 113–146, 2008.
Tinto, K. J., Padman, L., Siddoway, C. S., Springer, S. R., Fricker, H. A., Das, I., Tontini, F. C., Porter, D. F., Frearson, N. P., Howard, S. L., Siegfried, M. R., Mosbeux, C., Becker, M. K., Bertinato, C., Boghosian, A., Brady, N., Burton, B. L., Chu, W., Cordero, S. I., Dhakal, T., Dong, L., Gustafson, C. D., Keeshin, S., Locke, C., Lockett, A., O'Brien, G., Spergel, J. J., Starke, S. E., Tankersley, M., Wearing, M. G., and Bell, R. E.: Ross Ice Shelf response to climate driven by the tectonic imprint on seafloor bathymetry, Nat. Geosci., 12, 441–449, https://doi.org/10.1038/s41561-019-0370-2, 2019.
Uenzelmann-Neben, G., Gohl, K., Hochmuth, K., Salzmann, U., Larter, R. D., Hillenbrand, C.-D., Klages, J. P., PS104, S. T. of E., Afanasyeva, V., Arndt, J. E., Bickert, T., Bohaty, S. M., Dziadek, R., Ebermann, B., Ehrmann, W., Esper, O., Frederichs, T., Freudenthal, T., Gebhardt, C., Küssner, K., Kuhn, G., Najman, Y., Pälike, H., Riefstahl, F., Ronge, T., Scheinert, M., Pereira, P. S., Smith, J. A., Spiegel, C., Flierdt, T. V. de, and Zundel, M.: Deep water inflow slowed offshore expansion of the West Antarctic Ice Sheet at the Eocene-Oligocene transition, Commun. Earth Environ., 3, 36, https://doi.org/10.1038/s43247-022-00369-x, 2022.
Villa, G., Fioroni, C., Persico, D., Roberts, A.P., and Florindo, F.: Middle Eocene to late Oligocene Antarctic glaciation/deglaciation and Southern Ocean productivity, Paleoceanography, 29, 223–237, https://doi.org/10.1002/2013PA002518, 2014.
Warny, S., Askin, R. A., Hannah, M. J., Mohr, B. A. R., Raine, J. I., Harwood, D. M., Florindo, F., and Team, S. S.: Palynomorphs from a sediment core reveal a sudden remarkably warm Antarctica during the middle Miocene, Geology, 37, 955–958, https://doi.org/10.1130/g30139a.1, 2009.
Webb, P.-N.: Initial Report on Geological Materials Collected at RISP Site J9 1977-78, Ross Ice Shelf Project Management Office, University of Nebraska, Lincoln, 46 pp., 1978.
Webb, P.-N.: Initial Report on Geological Materials Collected at RISP Site J9 1978-79, Ross Ice Shelf Project Management Office, University of Nebraska, Lincoln, 127 pp., 1979.
Webb, P.-N.: Benthic foraminifera, in: Antarctic Cenozoic History from the CIROS-1 Drillhole, McMurdo Sound, edited by: Barrett, P. J., DSIR Bulletin 245, 99–118, 1989.
Webb, P.-N. and Strong, C. P.: Occurrence, stratigraphic distribution and palaeoecology of Quaternary foraminifera from CRP-1, Terra Antarctica, 5, 455–472, 1998a.
Webb, P.-N. and Strong, C. P.: Recycled Pliocene foraminifera from the CRP-1 Quaternary succession, Terra Antarctica, 5, 473–478, 1998b.
Webb, P.-N. and Strong, C. P.: Pliocene benthic foraminifera from CRP-2 (Lithostratigraphic Unit 2.2), Victoria Land Basin, Antarctica, Terra Antarctica, 7, 453–459, 2000.
Webb, P.-N. and Strong, C. P.: Foraminiferal biostratigraphy and palaeoecology in Upper Oligocene–Lower Miocene glacial marine sequences 9, 10, and 11, CRP-2/2A drill hole, Victoria Land Basin, Antarctica, Palaeogeogr, Palaeocl., 231, 71–100, https://doi.org/10.1016/j.palaeo.2005.07.036, 2006.
Webb, P.-N., Ronan, T. E., Jr, Lipps, J. H., and DeLaca, T. E.: Miocene glaciomarine sediments from beneath the southern Ross Ice Shelf, Antarctica, Science, 203, 435–437, https://doi.org/10.1126/science.203.4379.435, 1979.
Webb, P.-N., Leckie, R. M., and Ward, B. L.: Foraminifera (Late Oligocene), in: Antarctic Cenozoic History from the MSSTS-1 Drillhole, McMurdo Sound, Antarctica, edited by: Barrett, P. J., Bulletin in the Miscellaneous Series of the New Zealand Department of Scientific and Industrial Research, 237, 115–125, 1986.
Wrenn, J. H. and Beckman, S. W.: Maceral, Total Organic Carbon, and Palynological Analyses of Ross Ice Shelf Project Site J9 Cores, Science, 216, 187–189, https://doi.org/10.1126/science.216.4542.187, 1982.
Zachos, J. C., Breza, J. R., and Wise, S. W.: Early Oligocene ice-sheet expansion on Antarctica: Stable isotope and sedimentological evidence from Kerguelen Plateau, southern Indian Ocean, Geology, 20, 569–573, 1992.