the Creative Commons Attribution 4.0 License.
the Creative Commons Attribution 4.0 License.
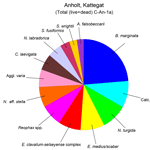
Assessing proxy signatures of temperature, salinity, and hypoxia in the Baltic Sea through foraminifera-based geochemistry and faunal assemblages
Jeroen Groeneveld
Helena L. Filipsson
William E. N. Austin
Kate Darling
David McCarthy
Nadine B. Quintana Krupinski
Clare Bird
Magali Schweizer
Current climate and environmental changes strongly affect shallow marine and coastal areas like the Baltic Sea. This has created a need for a context to understand the severity and potential outcomes of such changes. The context can be derived from paleoenvironmental records during periods when comparable events happened in the past. In this study, we explore how varying bottom water conditions across a large hydrographic gradient in the Baltic Sea affect benthic foraminiferal faunal assemblages and the geochemical composition of their calcite tests. We have conducted both morphological and molecular analyses of the faunas and we evaluate how the chemical signatures of the bottom waters are recorded in the tests of several species of benthic foraminifera. We focus on two locations, one in the Kattegat (western Baltic Sea) and one in Hanö Bay (southern Baltic Sea). We show that seawater Mn∕Ca, Mg∕Ca, and Ba∕Ca (Mn∕Casw, Mg∕Casw, and Ba∕Casw) variations are mainly controlled by dissolved oxygen concentration and salinity. Their respective imprints on the foraminiferal calcite demonstrate the potential of Mn∕Ca as a proxy for hypoxic conditions, and Ba∕Ca as a proxy for salinity in enclosed basins such as the Baltic Sea. The traditional use of Mg∕Ca as a proxy to reconstruct past seawater temperatures is not recommended in the region, as it may be overprinted by the large variations in salinity (specifically on Bulimina marginata), Mg∕Casw, and possibly also the carbonate system. Salinity is the main factor controlling the faunal assemblages: a much more diverse fauna occurs in the higher-salinity (∼32) Kattegat than in the low-salinity (∼15) Hanö Bay. Molecular identification shows that only Elphidium clavatum occurs at both locations, but other genetic types of both genera Elphidium and Ammonia are restricted to either low- or high-salinity locations. The combination of foraminiferal geochemistry and environmental parameters demonstrates that in a highly variable setting like the Baltic Sea, it is possible to separate different environmental impacts on the foraminiferal assemblages and therefore use Mn∕Ca, Mg∕Ca, and Ba∕Ca to reconstruct how specific conditions may have varied in the past.
- Article
(13091 KB) - Full-text XML
-
Supplement
(209 KB) - BibTeX
- EndNote
Shelf and fjord environments are known to be sensitive to and directly affected by global climate change. With ongoing climate change, seawater is warming, atmospheric circulation and precipitation patterns are changing, and – together with coastal circulation changes and human-induced eutrophication – these factors are driving decreased dissolved oxygen concentrations ([O2]) in many areas (Keeling and Garcia, 2002; Meier et al., 2011). Increasingly, hypoxic conditions ([O2]<2 mg L−1 or <1.42 mL L−1) occur in semi-enclosed basins like the Gulf of Mexico (Osterman et al., 2005; Platon et al., 2005), the Baltic Sea (Diaz and Rosenberg, 2008; Conley et al., 2011), and (Scandinavian) fjords (Gustafsson and Nordberg, 2000; Filipsson and Nordberg, 2004a, b).
Paleoenvironmental reconstructions have demonstrated that large environmental changes in the Baltic Sea have occurred, and how the system switched from fresh water lake stages (e.g., Ancylus Lake) at the end of the last deglaciation to more marine conditions during the Littorina Sea stage (∼8.5–3 ka; Björck, 1995, 2008; Jakobsson et al., 2007; Andrén et al., 2015; Kotthoff et al., 2017). Even during the saline Littorina Sea stage, variability in salinity remained high and several periods occurred when large areas of bottom waters became fully anoxic and even euxinic (Björck, 1995; Gustafsson and Westman, 2002; Zillén et al., 2008; Widerlund and Andersson, 2011; Jilbert and Slomp, 2013; Kotilainen et al., 2014; Lenz et al., 2015; Ning et al., 2016; Van Helmond et al., 2017).
To improve the reconstruction of past hydrographic conditions in the Baltic Sea region, it is necessary to validate how environmental proxies such as foraminifera-based trace metal ∕ Ca ratios respond to an environmental setting like the Baltic Sea where both intra- and interannual variations in water mass properties can be very large. Our aim is to explore the potential use of Mn∕Ca, Mg∕Ca, and Ba∕Ca in benthic foraminifera as proxies for dissolved oxygen concentration, bottom water temperature, and salinity in such a complicated setting. The advantage of analysing these three geochemical proxies on the same biotic carrier is that potential biases due to different biological circumstances, such as growth or depositional season, are minimized. Additionally, the proxy variations are fixed into the foraminiferal tests during calcification (as opposed to a secondary diagenetic imprint which may occur after deposition) and thus representative for the bottom water conditions for that particular period, while proxies based on bulk sediment composition may be altered over time by continuing (bio)geochemical processes within the sediment.
Manganese is a redox-sensitive element, present as dissolved Mn2+ in seawater but mostly precipitating as a Mn oxyhydroxide under oxic conditions. Under decreasing pore water oxygen conditions the Mn oxyhydroxide is reduced and the pore water Mn2+ concentration increases and diffuses in the sediment. Upwards diffusion occurs until either the Mn2+ escapes into the water column when bottom water conditions are hypoxic or re-precipitates as soon as free oxygen is encountered (Froelich et al., 1979; Canfield et al., 1993; Calvert and Pedersen, 1993; Burdige, 1993; Tribovillard et al., 2006). Pore water can become supersaturated with respect to Mn under anoxic conditions, resulting in inorganic precipitation of Mn carbonates like rhodochrosite. Thus, when benthic foraminifera live and precipitate calcium carbonate under hypoxic conditions, more Mn is available to be incorporated into their calcite tests so that Mn∕Ca in foraminifera may be used as a proxy for hypoxic conditions (Glock et al., 2012; Groeneveld and Filipsson, 2013; McKay et al., 2015; Koho et al., 2015; Petersen et al., 2018).
The increase in foraminiferal Mg∕Ca with higher seawater temperatures is a commonly used proxy, especially for planktonic foraminifera (e.g., Nürnberg, 2000; Elderfield and Ganssen, 2000). The reconstruction of bottom water temperatures using benthic foraminifera is more complicated as the carbonate chemistry often has a significant impact (Elderfield et al., 2006; Raitzsch et al., 2008). Additionally, the temperature gradients in the deep-sea and at higher latitudes like the Baltic Sea cover the lower end of normal temperature calibration ranges, resulting in relatively small temperature-driven changes in Mg∕Ca (Lear et al., 2002; Kristjánsdóttir et al., 2007; Skirbekk et al., 2016; Barrientos et al., 2018). In shallow settings like the Baltic Sea large seasonal variations in salinity and dissolved oxygen concentrations may also have an impact (Groeneveld and Filipsson, 2013).
Foraminiferal Ba∕Ca can be used as a salinity proxy in an enclosed, largely land-locked setting like the Baltic Sea. In areas in which salinity is directly influenced by river runoff, changes in Ba∕Casw are controlled by changes in the volume of fresh water input, and thus the approximate amount of precipitation in the hinterland (Lea and Boyle, 1991; Hönisch et al., 2011; Bahr et al., 2013). As river water contains considerably more barium than seawater (Turner et al., 1980), this leads to an inverse relationship between river runoff and salinity. This relationship has been used to reconstruct past variations in river runoff to the oceans based on foraminiferal Ba∕Ca (Hall and Chan, 2004; Weldeab et al., 2007; Bahr et al., 2013) and is further explored in this study.
Bottom water pH values in the Baltic can be very low (7.4), leading to partly dissolved tests even in living foraminifera (Charrieau et al., 2018). Due to the heterogeneous distribution of Mg in the tests of planktonic foraminifera (Sadekov et al., 2005; Hathorne et al., 2009), dissolution of the tests is known to decrease the Mg∕Ca and thereby underestimate reconstructed temperatures (Dekens et al., 2002; Regenberg et al., 2006). Specific dissolution effects on benthic foraminiferal Mg∕Ca are less well constrained, but as Mg in benthic foraminifera is also heterogeneously distributed (e.g., Raitzsch et al., 2011; Branson et al., 2013) it is likely that dissolution also affects benthic foraminiferal Mg∕Ca. Similarly, Mn and Ba are also heterogeneously distributed in the foraminiferal tests and often show a similar distribution as Mg (Hathorne et al., 2009; De Nooijer et al., 2017; Petersen et al., 2018), so that dissolution probably decreases the Mn∕Ca and Ba∕Ca too.
Salinity, dissolved oxygen concentration, the carbonate system, and temperature vary significantly on both intra- and interannual timescales in the bottom waters of the Baltic Sea. Salinity decreases rapidly over a short distance in the Danish straits from marine conditions in the Kattegat to brackish conditions in the central Baltic Sea (Baltic Proper). The large spatial gradient in salinity of the Baltic Sea is mainly controlled by the precipitation/evaporation balance of the surrounding land and its land-locked location. Occasional major Baltic inflow events do increase the salinity and oxygen concentrations in the deeper parts (Matthäus and Schinke, 1999; Mohrholz et al., 2015), but are generally only of short-lived temporal impact. Bottom water oxygen concentrations are often zero with H2S present in many of the deeper sub-basins of the Baltic Sea due to stagnant bottom waters and, in recent decades, increasing oxygen demand driven by human-induced eutrophication due to fertilizer input via rivers (Conley et al., 2011).
Benthic foraminifera have been used to successfully reconstruct paleo-conditions in the Skagerrak–Kattegat–Baltic region using changes in faunal assemblage composition (Conradsen et al., 1994; Jorissen et al., 1995; Filipsson and Nordberg, 2004a, b; Kotthoff et al., 2017), and foraminiferal test trace metal ∕ Ca ratios and stable isotope compositions (Kristensen and Knudsen, 2006; Anjar et al., 2012; Groeneveld and Filipsson, 2013; Butruille et al., 2016; Kotthoff et al., 2017; Dijkstra et al., 2018). Benthic foraminifera are traditionally identified using test morphology alone. However, morphospecies are not always well defined and the species concept can vary between different taxonomic schools (Haynes, 1992; Murray, 2007; Jones, 2013). In addition, in the case of abnormal tests or cryptic species, test morphology alone may be inadequate to distinguish between different species (e.g., Polovodova and Schönfeld, 2008; Pawlowski and Holzmann, 2014). Cryptic species are distinct genetic types that exhibit highly similar morphologies that are therefore labelled with the same morphospecies name. These cryptic species can also be ecologically distinct and hence in the reconstruction of paleo-conditions, should be considered as different biological species. A combination of approaches is therefore required for accurate taxonomic identification of cryptic species, including traditional faunal analyses using morphological traits in parallel with molecular characterization using DNA barcoding (Pawlowski and Holzmann, 2014; Darling et al., 2016). By combining these approaches, precise taxonomic assignment of foraminiferal species can be achieved for environmental proxy development and use.
In this study we explore how the differing water column chemistry between two contrasting locations (the saline Kattegat (salinity 20–32) and the brackish Hanö Bay (salinity 7–18) (Fig. 1) affects the composition of the benthic foraminiferal assemblages using morphological and molecular identification techniques, and how each site's different chemical signatures are incorporated into the foraminiferal tests of key species, including an exercise to determine the possible effects of seasonal variations.
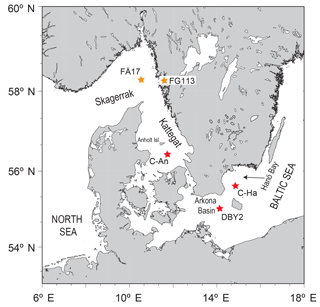
Figure 1Map of the study area indicating the locations of the sampling sites C-An (Kattegat, including C-An-1, C-An-2, DAn-1, and monitoring station Anholt-E), C-Ha (Hanö Bay; including C-Ha-1, C-Ha-2, DCHa-2, and monitoring station Hanöbukten), and DBY2 (Arkona Basin) (red stars; adapted from Groeneveld and Filipsson, 2013). Also shown are the locations of sites FÅ17 (Skagerrak) and FG113 (Gullmar Fjord) (orange stars; Groeneveld and Filipsson, 2013), which are used for comparison.
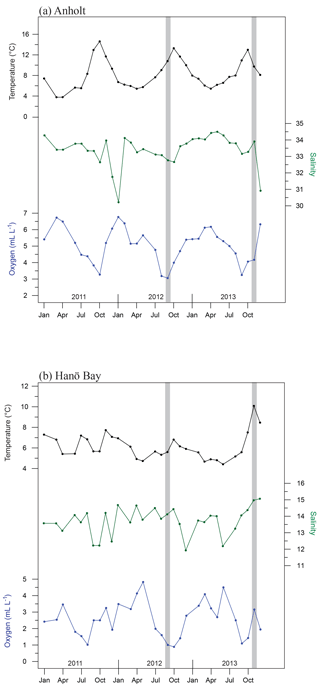
Figure 2Average bottom water conditions for 2011–2013, averaged per month, based on measurements by the Swedish Meteorological and Hydrological Institute (SMHI; temperature, salinity, oxygen) for (a) station Anholt-E, Kattegat (averaged for 30–60 m water depth) and (b) station Hanöbukten (averaged for >60 m water depth). Note that although an average value is shown, the steep gradient in dissolved oxygen occurs over this interval so that the deeper C-Ha-1 (71 m) may be hypoxic while C-Ha-2 (50 m) was oxic). Vertical grey bars indicate the time of water and foraminiferal sampling.
Long-term monitoring of water mass properties at stations near Anholt (Kattegat) and in Hanö Bay (Baltic Proper) is maintained by the Swedish Agency for Marine and Water Management (SWAM), through the Swedish Meteorological and Hydrological Institute (SMHI) and available via the SHARK hydrographic database (http://www.smhi.se, last access: August 2016). Monitoring data were used from the stations Anholt-E (56∘40′ N, 12∘07′ E) and Hanöbukten (Hanö Bay; 55∘37′ N, 14∘52′ E). Bottom water conditions (30–60 m water depth) in the Kattegat are very similar to open marine conditions, although relatively large variations can occur. Since the 1980s bottom water salinity varied between 32 and 34.5 (values similar to the Skagerrak and North Sea), and temperatures varied between 4 and 14 ∘C. Oxygen levels varied from 2 to 7 mL L−1, indicating that bottom water conditions have remained oxic for the last 30 years (SMHI). Bottom water conditions show similarly large variations on a seasonal timescale (temperature: 4–14 ∘C; salinity: 30–34; oxygen: 3–7 mL L−1; Fig. 2a) as over decadal timescales.
Continuous monitoring in Hanö Bay goes back to 1970 and interannual variations in bottom water (>60 m water depth) oxygen concentrations, temperature, and salinity (Fig. 2b; SMHI) are often related to the occurrences of major Baltic inflows of saline and oxygen-rich water from the North Sea (Matthäus and Schinke, 1999; Mohrholz et al., 2015). Accordingly, bottom water in Hanö Bay has been mostly hypoxic with occasional periods of increased oxygen concentrations. During several years, anoxic and euxinic conditions occurred, with H2S detected in the water column. Seasonal changes in Hanö Bay bottom water temperature (4–7.5 ∘C; although November 2013 recorded ∼10 ∘C) are less variable than in the Anholt area (Fig. 2b) mainly because the studied sites are at a greater water depth, but salinity (12–15) and oxygen concentration (1–5 mL L−1) show more comparable variability as in the Kattegat.
Samples were collected during two cruises with the R/V Skagerak; water samples and living foraminifera were collected in September 2012 to the Kattegat near Anholt (C-An-1 and C-An-2 stations, 30 m water depth) and Hanö Bay (C-Ha-1, 71 m water depth; C-Ha-2, 50 m water depth) (Table 1; Fig. 1), and in November 2013 from sites near Anholt (DAn-1, 61 m water depth) and in Hanö Bay (DCHa-2, 70 m water depth) (Table 1), and living foraminifera were collected from Arkona Basin (DBY2-1, 46 m water depth; Table 1; Fig. 1). Additionally, water samples from Hanö Bay from a cruise in June 2015 (C-Ha-3 station) were included in the interpretation (Table 1). At each location, CTD profiles were measured, and at selected water depths seawater was sampled in Niskin bottles (Table 1). Core top sediments were collected using a GEMAX twin barrel corer (modified Gemini corer, diameter 9 cm). The top 3 cm of the cores were sectioned into 1 cm slices and stained with Rose Bengal to identify foraminifera which were recently alive. The samples were preserved with ethanol. Foraminifera were picked from the first centimetre and separated into stained and non-stained specimens for faunal assemblages and geochemistry. During the same cruises, live foraminifera for culturing and molecular analyses were collected by multiple deployments of a box corer. The top sediment layers were retained for culturing experiments at the University of St. Andrews (to be reported elsewhere) and genetic characterization.
3.1 Foraminifer assemblages
Samples were processed according to standard laboratory methods using a 63 µm sieve for wet sieving of the samples, before drying and dry-picking (without splitting) the foraminifera from the size fraction >125 µm (Tables S1, S2 in the Supplement). Taxonomic identifications followed Loeblich and Tappan (1964) and Tappan and Loeblich (1988) for the genus level, and Feyling-Hanssen (1964), Feyling-Hanssen et al. (1971), and Murray and Alve (2011) for the species level. For original descriptions of the species, see Ellis and Messina (1940, and supplements up to 2013). To quantify the difference in diversity between Anholt and Hanö Bay the Shannon–Wiener index was calculated using the PAST software package (Hammer et al., 2001). Despite recent advances in the taxonomic identification of the elphidiids by Pillet et al. (2013) and Darling et al. (2016), this taxonomic group proved problematic to resolve across the large salinity gradients of the Baltic Sea based solely upon test morphology. Due to the difficulties of reliably distinguishing the gradational morphologies between the end-members of Elphidium clavatum and Elphidium selseyense, we have assigned all specimens to an E. clavatum-selseyense complex, which is subsumed in the morphospecies E. excavatum in other publications (e.g., Feyling-Hanssen, 1972). Ammonia beccarii and Ammonia falsobeccarii are commonly named as occurring in the area, but our molecular evidence shows that they should be identified as follows: A. beccarii in the Kattegat should be named Ammonia batava, molecularly identified as genetic type Ammonia T3S (Hayward et al., 2004); A. beccarii in Hanö Bay, also referred to as Ammonia aomoriensis, should be named Ammonia sp. (T6), molecularly identified as genetic type Ammonia T6 (Hayward et al., 2004); and A. falsobeccarii is molecularly identified as Ammonia T15 (Schweizer et al., 2011a).
3.2 Trace metal ∕ Ca and stable oxygen and carbon isotopes
The benthic foraminiferal species Ammonia falsobeccarii, Ammonia sp. (T6), Bulimina marginata, Cassidulina laevigata, the Elphidium clavatum-selseyense complex, Globobulimina turgida, Nonionella turgida, and Nonionellina labradorica were picked for the analysis of Mn∕Ca, Mg∕Ca, Ba∕Ca, and stable oxygen and carbon isotopes (δ18O and δ13C) based on their abundance in the samples. Foraminifera for geochemical analyses were picked from the size fractions 250–350 µm when available, otherwise from a wider size range. Between 8 and 80 specimens per sample were used for bulk trace metal ∕ Ca analyses and 5–20 specimens per sample for stable isotopes.
After gentle crushing of the foraminiferal tests, the samples were cleaned according to the standard cleaning protocol for foraminiferal Mg∕Ca analyses (Barker et al., 2003). Samples were dissolved in 0.5 mL 0.075 M QD HNO3 and centrifuged for 10 min (6000 rpm) to exclude any remaining insoluble particles from the analyses. Samples were diluted with Seralpur water before analysis with an ICP-OES (Agilent Technologies, 700 Series with autosampler ASX-520 Cetac and micro-nebulizer) at the MARUM, University of Bremen, Germany. Instrumental precision of the ICP-OES was monitored after every five samples by analysis of an in-house standard solution with a Mg∕Ca of 2.93 mmol mol−1 (long-term standard deviation of 0.026 mmol mol−1 or 0.88 %). A limestone standard (ECRM752-1) with a reported Mg∕Ca of 3.75 mmol mol−1 was analysed to allow inter-laboratory comparison (Greaves et al., 2008). The long-term average of the ECRM752-1 standard, which is routinely analysed twice before each batch of 50 samples in every session, is 3.78±0.066 mmol mol−1. Analytical precision based on three repetitions for each analysis for all species combined was 1.77 %, 0.12 %, and 3.58 % for Mn∕Ca, Mg∕Ca, and Ba∕Ca, respectively.
Stable oxygen (δ18O) and carbon (δ13C) isotope analyses on foraminiferal samples were performed on a Finnigan MAT 252 mass spectrometer equipped with an automatic carbonate preparation device at the MARUM,University of Bremen. The stable isotopic data are reported relative to the Vienna Peedee belemnite (VPDB) using the NBS19 standard. The standard deviation of the internal laboratory standard was 0.04 ‰ for δ18O and 0.02 ‰ for δ13C.
3.3 Foraminifer genetic types
The majority of specimens collected for DNA analyses were imaged under a scanning electron microscope (SEM) prior to genetic characterization to provide a link between morphology and genetic type for taxonomic designation (Darling et al., 2016). The methods used for genetic characterization are described in Darling et al. (2016) and are outlined here. Sediments from the top 1 cm of the box core employments from Anholt, Hanö Bay, and Arkona Basin were wet-sieved (63 µm) using seawater from the same location wherever possible and kept refrigerated at 4 ∘C prior to processing. Samples were examined using a stereomicroscope and individual specimens were picked using a fine brush, independent of size. Picked specimens were washed in filtered seawater and observed to determine whether they were alive. This was carried out by observing individual activity overnight in a Petri dish containing fine sediment. The live specimens identified were washed again and placed onto micropaleontological slides to air dry at room temperature. They could be kept for several weeks at room temperature before being mounted on stubs for gold coating and imaging using SEM (Philips XL30CP). Following imaging, they were removed from the stub and crushed into DOC buffer (Pawlowski, 2000) for DNA extraction.
DNA was amplified using the polymerase chain reaction (PCR). The primer pairs s14F3 and sB (Pawlowski, 2000) were used for the primary amplification of the 3′ terminal fragment (∼1000 bp) of the small subunit (SSU) rDNA and primer pairs s14F1 (Pawlowski, 2000) and J2 (Darling et al., 2016) for the secondary amplification. Where specimens were proving difficult to amplify, a shorter fragment (∼500 bp) was generated using primer pairs s14F1 and N6 (White et al., 1990) in the secondary amplification. Amplification products were purified using a Montage Gel Extraction Kit (Merck Millipore). Where there was evidence of multiple gene copies within an individual (intra-individual variation), PCR products were cloned using the pCR®-TOPO® Vector (Invitrogen). Sanger sequencing was performed using a BigDye Terminator v3.1 Cycle Sequencing Kit (Applied Biosystems) and an ABI 3730 DNA sequencer (Applied Biosystems).
Sequenced specimens of Elphidium and Ammonia were allocated a genetic type number and a morphospecies name according to previous molecular studies (Hayward et al., 2004; Schweizer et al., 2011a, b; Darling et al., 2016). In the study of Hayward et al. (2004), Ammonia genetic types were defined by large subunit (LSU) rDNA sequencing and in the studies of Schweizer et al. (2011a, b) by both LSU and SSU rDNA sequencing. For Elphidium, the morphological profile descriptions of the genetic types were used as the basis for their taxonomic designations (Darling et al., 2016). Sequences were edited in ChromasPro v1.5 (Technelysium Pty Ltd.) and compared with the Basic Local Alignment Search Tool (BLAST; Madden, 2002) to identify the genetic types present in the studied material. Our sequences were then manually aligned in BioEdit v7.0.9.0 (Hall, 1999) with sequences of the studied genera (i.e., Ammonia, Bulimina, Cassidulina, Elphidium and Nonionellina) previously deposited in the GenBank database (http://www.ncbi.nlm.nih.gov/genbank/, last access: June 2016).
3.4 Seawater properties
Seawater temperature, salinity, and dissolved oxygen profiles were measured through the water column using a CTD (Sea-Bird SBE 91). Separate seawater samples were collected in 20 mL glass bottles for element, stable oxygen isotope, and salinity analyses, respectively, taken from the standard Niskin (10 L) collection system attached to the CTD. The bottles were rinsed with water from the current sampling depth and a half-bottle volume was allowed to overflow before sampling. The sample bottles for all analyses were tightly capped and sealed with Parafilm® and refrigerated until analysis. High precision salinity measurements were performed on water samples collected in 2012 at the Marine Scotland Science Laboratories, Aberdeen using a Guideline Portasal, calibrated against IAPSO Standard Seawater (McKenna et al., 2016). Samples for element concentrations were acidified with 0.2 mL of 65 % QD HNO3 on board and diluted 10 times with Seralpur water before analysis with an ICP-OES (Agilent Technologies, 700 Series with autosampler ASX-520 Cetac and micro-nebulizer) at the MARUM, University of Bremen. The IAPSO and CRM standards were analysed to monitor the measurements. Analytical precision was 5.76 %, 0.13 %, and 1.77 % for Mn∕Casw, Mg∕Casw, and Ba∕Casw, respectively. Reproducibility based on re-measurement of all water samples (n=15) was 3.21 %, 0.08 %, and 2.17 % for Mn∕Casw, Mg∕Casw, and Ba∕Casw, respectively. Water samples were sealed in air-tight glass water bottles and analysed later for δ18O content on a Finnigan Delta plus XP gas source mass spectrometer, coupled to a GasBench II preparation system, at the School of Geography and Sustainable Development, University of St. Andrews. The δ18O measurements were based on 1 mL volumes, determined via CO2 equilibration. The mean precision of the triplicate analysis was ±0.04 ‰ relative to Vienna Standard Mean Ocean Water (VSMOW2). Multiple samples were measured in triplicate and three internal standard waters (calibrated to VSMOW2) were analysed every 10 samples.
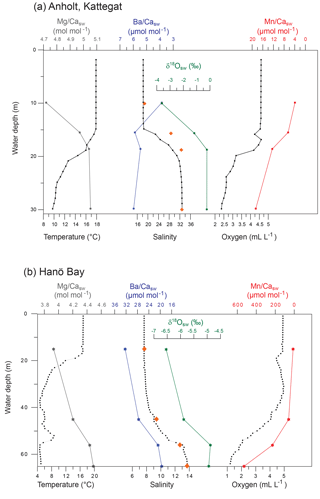
Figure 3Characteristic water column data for (a) Anholt (station C-An-2; September 2012) and (b) Hanö Bay (station C-Ha-1; September 2012) showing CTD data (small black circles), δ18Osw, Minisal salinity (orange diamonds), and Me∕Casw data (coloured stars) with water depth (Mg∕Casw, Mn∕Casw, Ba∕Casw).
4.1 Water column chemistry
4.1.1 Kattegat
The water column at the Anholt site (Kattegat) showed decreasing temperatures from 17.9 ∘C at the surface to 9.6 ∘C near the bottom in September 2012 (Fig. 3). Salinity increased from 18.4 at the surface to 32.9 near the bottom. The water column was oxic at all depths, but oxygen concentrations decreased from 4.52 mL L−1 at the surface to 2.32 mL L−1 near the bottom. These changes in water mass conditions were also reflected in the concentrations of calcium, magnesium, barium, manganese, and their respective ratios to calcium (Fig. 3; Table 1). Magnesium and calcium concentrations increased with increasing salinity, while the manganese concentration increased with decreasing dissolved oxygen to 12.4 µg L−1 ( µmol mol−1) near the bottom. The stable oxygen isotope composition of the water (δ18Osw) increased parallel with salinity from −3.62 ‰ at 10 m water depth to −0.23 ‰ near the bottom (Fig. 3). In November 2013, temperatures decreased from 11.5 ∘C at the surface to 9.2 ∘C near the bottom, salinity increased from 24 at the surface to 34 near the bottom, and oxygen decreased from 6.4 mL L−1 at the surface to 4.4 mL L−1 near the bottom; the metal concentrations were similarly linked to the hydrographic properties as in 2012 (Table 1).
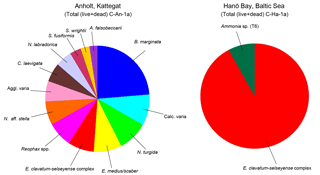
Figure 4Distribution of benthic (live and dead) foraminifera for the Kattegat (station C-An-1a) and Hanö Bay (station C-Ha-1a). Only species with an abundance >1 % are shown. Noticeable is the presence of only three species in Hanö Bay (Reophax spp. = 0.5 %, not shown), while the species diversity in the Kattegat near Anholt is much higher.
4.1.2 Hanö Bay
The water column in Hanö Bay was distinctly different from the Kattegat (September 2012). Temperatures decreased from 16.7 ∘C at the surface to 5.0 ∘C near the bottom, while salinity was much lower (7.7) near the surface, increasing to 13.7 near the bottom (Fig. 3; Table 1). Oxygen concentrations decreased from oxic conditions near the surface (4.87 mL L−1) to hypoxic conditions in the deeper water (1.21 mL L−1). These different conditions were also reflected in the chemical composition of the water column. Calcium and magnesium concentrations were on average 2–3 times lower than in the Kattegat, while the barium concentration was about 3 times higher (∼12 µg L−1; Table 1). Due to hypoxic conditions deeper in the water column, manganese concentrations increased to 138.7 µg L−1. Element ∕ Ca ratios also varied considerably (Fig. 3). Mg∕Casw was as low as 3.93 mol mol−1 at the surface with low salinity and 4.49 mol mol−1 near the bottom with higher salinity; in comparison, open ocean Mg∕Casw values are typically reported in the range of 5.1–5.2 mol mol−1. Ba∕Casw decreased from 32.3 µmol mol−1 at the surface to 19.5 µmol mol−1 near the bottom, showing a negative trend with increasing salinity. Mn∕Casw rapidly increased with decreasing dissolved oxygen concentrations from 12.33 µmol mol−1 at the surface to 529 µmol mol−1 near the bottom (Fig. 3). The stable oxygen isotope composition of the water (δ18Osw) increased parallel with salinity from −6.54 ‰ at 15 m water depth to −4.88 ‰ near the bottom (Fig. 3).
The water column in June 2015 was very similar to September 2012, but November 2013 showed indications of a possible inflow of saltier and more oxygenated water from the Kattegat. Bottom water salinity was 18 in comparison to ∼14 during the other cruises, and bottom water oxygen concentrations remained nearly oxic ([O2]=2.34 mL L−1) in comparison to hypoxic conditions in 2012 and 2015 ( mL L−1) (Table 1) with slightly lower Ba and Mn concentrations.
4.2 Foraminifer assemblages
Summary faunal counts of the living and dead (and total) foraminiferal assemblages at Anholt and Hanö Bay are reported in Tables S1 and S2, and summarized in Fig. 4.
4.2.1 Morphological assemblages
Kattegat
The foraminiferal assemblage of Anholt (station C-An-1) consisted of a diverse assemblage (Shannon–Wiener index = 2.5) dominated by Bulimina marginata (21 %; Fig. 4; Tables S1, S2). Several taxa provided 2–8 % of the assemblage including the hyaline Nonionella turgida, Elphidium clavatum-selseyense complex, Nonionella aff. stella, Cassidulina laevigata, Nonionellina labradorica, Stainforthia fusiformis, Ammonia falsobeccarii, and the agglutinated taxa Eggerelloides medius/scaber, Reophax spp., and Spiroplectammina wrightii.
Hanö Bay
The foraminiferal assemblage in the low salinity, low oxygen environment of Hanö Bay (Station C-Ha-1, 71 m water depth) was considerably different from the Kattegat, and was characterized by low diversity (Shannon–Wiener index = 0.3) and high dominance of specimens belonging to the Elphidium clavatum-selseyense complex (91 %) and a few, generally etched and broken, specimens of Ammonia sp. (T6) (∼8 %) and Reophax spp. (<1 %) (Fig. 4; Tables S1, S2).
4.2.2 Foraminifer genetic types
Molecular characterization was carried out on foraminifera collected from sampling stations at the Anholt (C-An-1 and DAn-1), Arkona Basin (DBY2-1), and Hanö Bay (C-Ha-1 and C-Ha-2) sites (Fig. 1). From a total of 249 live specimens picked and SEM imaged, 131 specimens were successfully genetically characterized (Tables 2, S3) and their sequences deposited in the GenBank database (accession numbers KX962726-KX962802 and KX963042-KX963062, previously published in Darling et al., 2016; and MF170098-MF170141, this study). Sequences were identified using BLAST and manually aligned against sequences of the same genera available in GenBank (see the Methods section). Genetic type designations for Elphidium were based on Darling et al. (2016) and for Ammonia on Hayward et al. (2004) and Schweizer et al. (2011a, b).
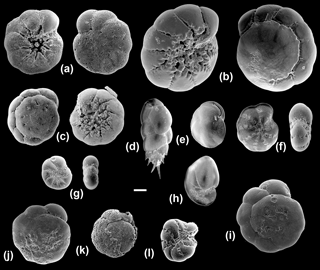
Figure 5Scanning electron microscope images of the benthic foraminiferal species sequenced in this study (all imaged specimens were successfully sequenced except for BA39). (a) Ammonia T6 (Ammonia sp. (T6); BA76), umbilical and spiral views; (b) Ammonia T3S (A. batava; BA38), umbilical and spiral views; (c) Ammonia T15 (A. falsobeccarii; BA39), umbilical and spiral views; (d) Bulimina marginata (BA29); (e) Cassidulina laevigata (BA56); (f) Elphidium S4 (E. clavatum; BA2), side and apertural views; (g) Elphidium S7 (E. albiumbilicatum; BA127), side and apertural views; (h) Nonionellina labradorica (BA37); (i–k) Specimens from the Hanö Bay site with traces of dissolution: Ammonia T6 (Ammonia sp. (T6); BA75, BA80, BA146); (l) abnormal test: Elphidium S4 (E. clavatum; BA87) side view. Scale bar: 100 µm.
Kattegat
Of the 77 specimens processed, 32 were successfully sequenced from the two stations near Anholt (Fig. 1; Table 2). Elphidium and Ammonia specimens were rare and their amplification success was relatively poor at this site. However, of the eight Ammonia processed, two morphologically distinct specimens of Ammonia were successfully sequenced and identified as genetic types Ammonia T3S (A. batava) and Ammonia T15 (A. falsobeccarii), respectively (Fig. 5b, c). Of the five specimens of Elphidium processed, only three were successfully sequenced. They were all identified as the genetic type Elphidium S4, corresponding to the morphospecies Elphidium clavatum (Fig. 5f; Darling et al., 2016).
Three other rotaliids were morphologically identified (Fig. 5d, e, h) and successfully sequenced at the Anholt stations: B. marginata (n=13), C. laevigata (n=13), and N. labradorica (n=1; Table 2). For the genus Bulimina, the partial SSU rDNA sequences of 13 individuals morphologically identified as B. marginata in our sample set had 98 %–99 % identity using BLAST to GenBank sequences from specimens identified as B. marginata from the Skagerrak and New Zealand (Schweizer et al., 2005; Holzmann and Pawlowski, 2017).
For the genus Cassidulina, partial SSU rDNA sequences from 13 individuals sampled at Anholt had 98 %–99 % identity using BLAST to GenBank sequences from specimens collected in the Oslo Fjord, which were identified as C. laevigata (Schweizer et al., 2005, 2009). However, they differ from the GenBank sequences HG966253-HG966296, retrieved from specimens collected in the Faroe Islands, which were also identified as C. laevigata (Weber and Pawlowski, 2014). This implies that there may be cryptic diversity within this group. A more in-depth study including morphometric analysis and comparison with type material, as advocated by Roberts et al. (2016), would be needed to investigate the different Cassidulina found in northern Europe and link the DNA sequences to taxonomic names.
The sequence belonging to the Nonionellina specimen BA37 is very short (189 bp) and only of medium quality. Using BLAST, it is 91 % similar to sequences from specimens of N. labradorica collected in the Skagerrak, Svalbard (Schweizer et al., 2005, 2008, 2009) and the White Sea (Holzmann and Pawlowski, 2017). Nevertheless, N. labradorica is still the closest known taxon identified in GenBank and will be retained here because it corresponds to the morphological identification of the sequenced specimen BA37 (Fig. 5h). The low similarity score of the sequence is most probably an artefact, due to the very low quality and length of the sequence.
Hanö Bay
From a total of 102 specimens, 92 were successfully sequenced from Hanö Bay (Fig. 1; Table 2). Amplification success rate was surprisingly high (84 %), even though many tests at this low temperature and salinity site were heavily damaged, abnormal, or dissolved (Fig. 5i–l); Charrieau et al. (2018) suggested seasonal fluctuations in carbonate chemistry and salinity as the most likely cause for test dissolution in the area. Of the 20 specimens of Ammonia processed, 18 were successfully sequenced and all were found to be genetic type Ammonia T6 (Ammonia sp. (T6); Fig. 5a). This genetic type was not identified in either Anholt or the Arkona Basin, although numbers of Ammonia sequenced at these sites were very low. However, the genetic types Ammonia T3S (A. batava) and Ammonia T15 (A. falsobeccarii) identified near Anholt were not found in Hanö Bay. Of the 82 specimens of Elphidium processed in Hanö Bay, 74 were successfully sequenced. They were identified as the genetic types Elphidium S4 (n=70) and Elphidium S7 (n=4) which are linked to the morphospecies E. clavatum and E. albiumbilicatum, respectively (Fig. 5f, g). Elphidium albiumbilicatum was only genetically identified at station C-Ha-2.
Arkona Basin
Additionally, 7 specimens were sequenced from a total of 70 at the Arkona Basin site, southwest of Hanö Bay (Fig. 1; Table 2). A single live specimen of Ammonia, morphologically identified as A. falsobeccarii was found in the non-sequenced sample set, which failed to amplify. Of the seven Elphidium specimens successfully amplified, three were identified as genetic type Elphidium S4 and four as genetic type Elphidium S7. Elphidium S4 was linked to the morphospecies Elphidium clavatum and Elphidium S7 to morphospecies Elphidium albiumbilicatum (Fig. 5g).
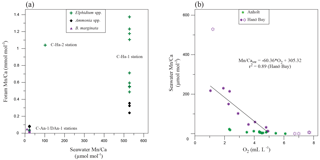
Figure 6(a) Mn∕Ca in different species of foraminifera (Elphidium spp., Ammonia spp., B. marginata) from Anholt and Hanö Bay in comparison with Mn∕Casw. Note that no results from the deeper infaunal species N. labradorica and G. turgida were included.; (b) Mn∕Casw in comparison with dissolved oxygen for Anholt (green; stations C-An-1, C-An-2, DAn-1) and Hanö Bay (purple; stations C-Ha-1, C-Ha-2, C-Ha-3, DCHa-2). Lighter purple symbols were not included in determining the relation between dissolved oxygen and Mn∕Casw.
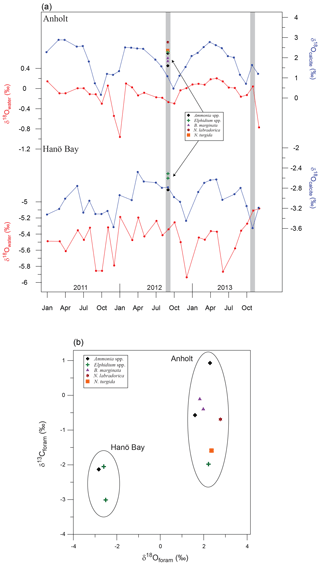
Figure 7(a) Calculated δ18Osw for Anholt and Hanö Bay based on instrumental water salinity recordings (Fröhlich et al., 1988). Only data from water depths greater than 30 m (Anholt) and 60 m (Hanö Bay) were used. Equilibrium δ18Ocalcite was calculated combining δ18Osw and instrumental temperatures for Anholt and Hanö Bay (Epstein et al., 1953). Grey vertical bars indicate the time of sampling; coloured symbols indicate the analysed δ18Ocalcite for different species in comparison with equilibrium δ18Ocalcite. Note that the scales for the different curves are not the same and that they are not plotted in reverse; (b) δ18Ocalcite vs. δ13Ccalcite for the different species from Hanö Bay and Anholt.
Table 3Geochemical results for element ∕ Ca and stable isotopes for foraminiferal species analysed at Anholt and Hanö Bay in comparison with water column properties. Note that all analyses were performed on samples consisting of multiple specimens.
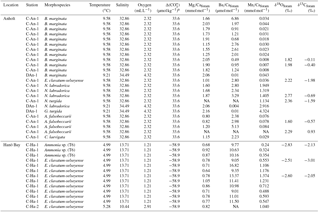
a Carbonate saturation state for stations C-An-1, C-Ha-1, and C-Ha-2 was calculated using CO2 SYS (Pierrot et al., 2006) with the constants of Mehrbach et al. (1973) and pH on the seawater scale, and based on measurements taken during the DISCO cruise (Charrieau et al., 2018).
4.3 Foraminifer geochemistry
4.3.1 Kattegat
Trace metal ∕ Ca ratios were analysed on B. marginata (n=12), E. clavatum-selseyense complex (n=1), N. labradorica (n=4), G. turgida (n=1), N. turgida (n=1), A. falsobeccarii (n=3), and C. laevigata (n=1) (Table 3). Mn∕Ca values varied between 0.01 and 4.32 mmol mol−1. The highest Mn∕Ca values are for N. labradorica (1.90±0.73 mmol mol−1), G. turgida (4.32 mmol mol−1), and N. turgida (1.13 mmol mol−1) (Table 3), while for the other species all values were <0.1 mmol mol−1 (Fig. 6a). Mg∕Ca ratios varied between 0.80 and 2.16 mmol mol−1 with the lowest average value for A. falsobeccarii of 0.94±0.22 mmol mol−1 and highest Mg∕Ca for G. turgida (2.16 mmol mol−1) (Table 3). Ba∕Ca varied between 0.004 and 6.86 µmol mol−1. Average Ba∕Ca for all species was 2.08±1.63 µmol mol−1 (Table 3). Stable oxygen and carbon isotopes were analysed on seven samples (B. marginata (2), E. clavatum-selseyense complex, N. labradorica, N. turgida, and A. falsobeccarii (2)) with δ18O ranging between 1.60 ‰ (A. falsobeccarii) and 2.77 ‰ (N. labradorica), and δ13C ranging between −1.98 ‰ (E. clavatum-selseyense complex) and +0.93 ‰ (A. falsobeccarii) (see Table 3 and Fig. 7 for species-specific values).
4.3.2 Hanö Bay
Trace metal ∕ Ca were analysed on Ammonia sp. (T6) (n=3) and on the E. clavatum-selseyense complex (n=10) (Table 3). Mn∕Ca values varied between 0.24 and 1.37 mmol mol−1. Average Mn∕Ca is lowest for Ammonia sp. (T6) (0.31±0.06 mmol mol−1) and 0.88±0.34 mmol mol−1 for the E. clavatum-selseyense complex (Fig. 6a). Mg∕Ca values varied between 0.64 and 1.05 mmol mol−1 with an average value for both species of 0.80±0.11 mmol mol−1 (Table 3). Ba∕Ca values varied between 9.01 and 16.82 µmol mol−1 with an average for both species combined of 11.09±2.19 µmol mol−1 (Table 3). Stable oxygen and carbon isotopes were analysed on Ammonia sp. (T6) ( ‰; ‰) and the E. clavatum-selseyense complex ( ‰ and −2.60 ‰; ‰ and −3.01 ‰) (Table 3, Fig. 7).
5.1 Water column differences between the Kattegat and Hanö Bay (Baltic Sea)
The manganese concentration in the water column in the Kattegat and Hanö Bay reflects the dissolved oxygen concentration that determines the redox conditions, so that with decreasing oxygen the manganese increases (Figs. 3; 6). We used the data from Hanö Bay, where the change in Mn2+ and dissolved oxygen is much larger than in the Kattegat to establish a relationship between Mn∕Casw and dissolved oxygen (Fig. 6b). Data points from the surface layers were excluded as under these conditions (O2>5 mL L−1) the Mn concentration is not determined by the redox potential but rather by supply of manganese from rivers and the atmosphere (Burton et al., 1988); and one outlier with a µmol mol−1 was also excluded. The resulting relation between dissolved oxygen and Mn∕Casw ratio can be expressed as a linear expression (Fig. 6b):
The much higher manganese concentrations in Hanö Bay than in the Kattegat suggest that Mn2+ from manganese oxides, which are reduced in the sediment, is able to escape into the hypoxic water column in Hanö Bay (Froelich et al., 1979; Burdige, 1993). Unfortunately no pore water manganese concentrations are available to confirm this, but bottom water manganese concentrations in Hanö Bay are similar to typical hypoxic pore water concentrations from Gullmar Fjord on the Swedish west coast (Goldberg et al., 2012) or the oxygen minimum zone of Peru (Scholz et al., 2011).
Alkalinity is an important component of the carbonate system, and in the southern Baltic Sea it is comparably high (1600–1800 µmol L−1), though still considerably lower than in the open ocean (>2200 µmol L−1; Hjalmarsson et al., 2010). The four largest rivers in the Baltic catchment (Neva, Vistula, Daugava, and Odra) all drain through areas with carbonate-rich bedrock as well as carbonate-rich Quaternary deposits. Weathering of these rocks increases both alkalinity and calcium in the southern part of the Baltic Proper. An increase in alkalinity and Ca concentrations since the 1950s was interpreted as a result of increasingly acidic rainfall in the catchment areas (Ohlson and Anderson, 1991; Dyrssen, 1993; Kremling and Wilhelm, 1997). Despite the higher calcium vs. chlorinity ratio (an expression for salinity) compared with open ocean ratios, calcium concentrations in the Baltic Sea still decrease with decreasing salinity (Grasshoff and Voipio, 1981; Löfvendahl et al., 1990).
The magnesium concentration also decreases with lower salinity (Table 1), yet the ratio of magnesium vs. chlorinity remains similar to the open ocean ratio (Grasshoff and Voipio, 1981). Accordingly, Mg∕Casw in the Baltic Sea decreases with decreasing salinity, i.e., a decrease of ∼10 % when comparing bottom water Mg∕Casw in the Kattegat vs. Hanö Bay (5.05 vs. 4.49 mol mol−1; Fig. 3; Table 1). This decline in Mg∕Casw is also expected to result in a decrease in foraminiferal Mg∕Ca (Segev and Erez, 2006; Raitzsch et al., 2010; Evans and Müller, 2012).
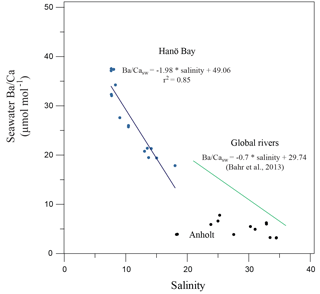
Figure 8Ba∕Casw in comparison with salinity for Hanö Bay and the Kattegat. A composite for major global river mouths (Bahr et al., 2013) is also shown. A correlation exists for Hanö Bay that is steeper than the global river composite, while no correlation exists for the Kattegat.
Barium concentrations in the Kattegat show no Ba∕Casw relationship with salinity because river runoff is not the main factor controlling salinity due to the open connection to the Skagerrak, North Sea, and the North Atlantic (Figs. 3, 8). Barium concentrations and Ba∕Casw in Hanö Bay, however, do vary with changes in salinity (Fig. 3; Table 1). The Ba concentration increases when salinity decreases because Ba in the Baltic is mainly supplied by river runoff, which contains more Ba than Baltic Sea water (27–95 µg L−1; Salminen, 2005). An inverse relationship exists through the water column in Hanö Bay between Ba∕Casw and salinity (Fig. 8):
This relationship is steeper than a compilation of Ba∕Casw samples from the mouths of global major rivers (Congo, Ganges, Amazon) given by Bahr et al. (2013, and references therein), although the Bahr et al. study covered a higher range of salinity (21–36) than in Hanö Bay (8–18; Fig. 8).
A potential source of contamination for dissolved barium is the presence of barite (BaSO4) both in the water column and in sediments. In the Baltic Sea, however, it appears that barite formation is restricted to surface waters under direct influence of river inflow, and no significant barite formation takes place in the sediments (Bernard et al., 1989; Ingri et al., 1991). The barium which is nevertheless included into suspended particles is often scavenged by Mn oxyhydroxides and not affecting the Ba∕Ca ratio (Boström et al., 1982).
5.2 Foraminifer assemblages and genetic types
The faunal composition near Anholt is very similar to those found in normal shallow-marine conditions in the Kattegat and Skagerrak (Feyling-Hanssen et al., 1971; Nordberg and Bergsten, 1988; Conradsen et al., 1994; Alve and Murray, 1999; Erbs-Hansen et al., 2012; Charrieau et al., 2018). The completely different faunal composition in Hanö Bay can be explained by the much lower salinity and, especially in the deeper station, hypoxic conditions as was also shown by Charrieau et al. (2018). Diversity is considerably lower in Hanö Bay with only three taxa (E. clavatum-selseyense complex, Ammonia sp. (T6), and Reophax spp.) identified in the assemblage analysis (Fig. 4; Tables S1, S2). Interestingly, the molecular analyses also identified E. albiumbilicatum in Hanö Bay (station C-Ha-2) and Arkona Basin (station DBY2). The morphologically-based faunal analysis (Rose Bengal stained samples) was conducted on samples from the deeper, more hypoxic station C-Ha-1, which may explain why E. albiumbilicatum was not identified there as it is not directly associated with low oxygen conditions (Murray, 2006; Darling et al., 2016). Taxonomic designations for members of the Ammonia and Elphidium genera can be highly challenging, but molecular characterization in combination with taxonomic evidence from the literature substantially improves our understanding of their classification, allowing the designation of morphospecies names when distinct morphological characters are identified.
Representatives of the genus Ammonia are distributed around the coasts of the North Sea, the Skagerrak, and the Kattegat, but it was suggested that Ammonia may be absent in recent sediments from the Baltic Sea (Murray, 2006), except in the southwestern region around Kiel, where it is thought to be a recent arrival (Lutze, 1965; Polovodova and Schönfeld, 2008; Pawlowski and Holzmann, 2008; Schweizer et al., 2011b) and the Belt seas (Lutze, 1965). Recent work showed the repeated occurrence of A. beccarii over the past 7500 years in the southwestern Baltic (Little Belt; Kotthoff et al., 2017). Previous molecular studies have shown that at least four genetic types (Ammonia T3S, Ammonia T15, Ammonia T6 and Ammonia T1) inhabit these regions (Hayward et al., 2004; Schweizer et al., 2011a, b).
In the current study, Ammonia specimens were relatively rare at the sampled water depths of ∼30 m at the Anholt stations and ∼50–70 m at the Hanö Bay stations (Fig. 4), and only three of the four regionally recognized genetic types (Ammonia T3S, Ammonia T15, Ammonia T6) were identified. Genetic type Ammonia T1 was found in the eastern Skagerrak by Hayward et al. (2004) as a shallow water dweller inhabiting a micro-tidal marsh (Holzmann and Pawlowski, 2000), and not found at the depths sampled in this study. However, specimen numbers were particularly low and we cannot exclude the possibility that it also occurs in the Kattegat.
Ammonia T3S (A. batava; Hayward et al., 2004) is a large and heavily ornamented species (Fig. 5b), which can be discriminated morphologically from the other Ammonia morphospecies. It has previously been identified in the Skagerrak, occurring in open marine habitats and the seaweeds of rocky shores (Hayward et al., 2004), and now in this study at the Anholt station (∼30 m water depth; Table 2).
Ammonia T15 (A. falsobeccarii; Schweizer et al., 2011a) has previously only been sequenced in the Bay of Biscay (140 m water depth) and Rhone pro-delta (60 m water depth) (Schweizer et al., 2011a) and now in this study at 30 m water depth near Anholt (Table 2). Ammonia falsobeccarii (Fig. 5c) can be discriminated morphologically from other Ammonia species, as it has secondary openings on the spiral side (Schweizer et al., 2011a). It has been morphologically identified in the same subtidal marine habitats as Ammonia T3S (Schweizer et al., 2011a) and was imaged in Hayward et al. (2004), indicating its presence in the Skagerrak.
In Europe, Ammonia T6 was previously sequenced at the Dutch, German, and Danish coasts of the North Sea and the Kiel Fjord in the southwestern Baltic Sea (Hayward et al., 2004; Schweizer et al., 2011b) and now in this study in Hanö Bay, Baltic Sea (Table 2). Ammonia T6 (Fig. 5a, i, j, k) is very difficult to discriminate morphologically from the genetic types Ammonia T1 and Ammonia T2 (Hayward et al., 2004). However, using a 36-character morphometric analysis, it was possible to discriminate T6 from the other genetic types and Ammonia T6 was allocated the morphospecies name Ammonia aomoriensis by Hayward et al. (2004). T6 has different environmental preferences from T3S and T15, living in tidal flats, marshes, and brackish lakes (Hayward et al., 2004). In this study, it has been identified in the low salinity environment of the Baltic Sea at a water depth between 50 and 70 m, which indicates that it has a particular tolerance to low salinity environments or even an affinity for them. In addition, this species seems highly tolerant of hypoxic conditions (Nardelli et al., 2014; Petersen et al., 2016). The tests of the sequenced specimens were often heavily dissolved (Fig. 5), yet they were still alive, as demonstrated by the success in sequencing (Schweizer, 2015).
Representatives of the genus Elphidium are present in both the Skagerrak and the Baltic Sea (Murray, 2006). Genetic type Elphidium S4 (Elphidium clavatum; Darling et al., 2016) is known as a brackish species, mainly occurring in Arctic regions, where it is often dominant in glacier-proximal environments, but is also found in the Baltic and southern Scandinavian fjords where it inhabits the deeper parts of the basins which are often oxygen-depleted (Murray, 2006; Darling et al., 2016). Another taxonomic issue is the frequent inclusion of E. clavatum within the morphospecies Elphidium excavatum, from which it is often regarded as an ecophenotype or a subspecies (e.g., Feyling-Hanssen, 1972). However, molecular studies have shown that E. clavatum has full species rank (Schweizer et al., 2011b; Pillet et al., 2013; Darling et al., 2016). Elphidium S4 (Elphidium clavatum) is the only genetic type identified at all three sites of Anholt, Arkona Basin and Hanö Bay, which indicates it is a euryhaline species (Darling et al., 2016).
Genetic type Elphidium S7 (Elphidium albiumbilicatum, Darling et al., 2016) is a high-latitude species found in Arctic and boreal waters as far south as the English Channel, and in the low-salinity Baltic Sea (Darling et al., 2016). It is an intertidal to shallow subtidal species whose occurrence is probably controlled by salinity (Murray, 2006). Elphidium S7 (Fig. 5) was found in Arkona Basin and Hanö Bay but not in Anholt, which would confirm its preference for more brackish waters.
The other rotaliid taxa, B. marginata, C. laevigata and N. labradorica (Fig. 5), molecularly identified within the study, were only found at the Anholt station, which is most similar to open marine conditions. The molecular identification analyses thus confirm the conventional morphology-based faunal analyses that benthic foraminiferal biodiversity is much higher at the Anholt station, where typical shelf sea species common in the Skagerrak and the North Sea are found (Murray, 2006).
5.3 Foraminiferal geochemistry: stable oxygen and carbon isotopes
Stable oxygen and carbon isotopes were analysed on a limited number of samples due to low foraminiferal abundances (Table 3). The average δ18O difference between samples with the same species analysed both from Kattegat and Hanö Bay was ∼4.8 ‰, as is also reflected in the δ18O vs. δ13C plot (Fig. 7). The large difference in δ18O is primarily due to the large difference in δ18Osw between the two sites (Figs. 3, 7). The difference between calculated δ18Oeq and measured δ18O ranges between 0.5 ‰ (A. falsobeccarii) and 1.6 ‰ (N. labradorica) depending on species for the shallow water Anholt site (C-An-1). Apart from Elphidium ( ‰ to −0.8 ‰ offset from equilibrium), all analysed species are calcifying close to equilibrium with δ18Osw (McCorkle et al., 1997; Polyak et al., 2003; Bauch et al., 2004; Schmiedl et al., 2004; Toyofuku et al., 2011). This implies that the foraminifera near Anholt may have calcified during a different time of the year. Few studies have been conducted to date on δ18O in Baltic foraminifera. Schönfeld and Numberger (2007) analysed living E. excavatum from the Kiel Bight with a salinity of 17–21 and a temperature measured between 4 and 10 ∘C. Their δ18O values (−0.6 ‰ to −1.1 ‰) lie between those we present from the Kattegat and Hanö Bay.
The carbon isotopic composition varies between the different species as a result of differences in habitat depth and site location. At the more open-marine Anholt location, δ13C values vary between the different species according to their respective habitat depths, with more negative values corresponding to the deeper infaunal species N. turgida, N. labradorica and possibly Elphidium spp. (Fig. 7b; McCorkle et al., 1997; Schmiedl et al., 2004). The δ13C data from Hanö Bay are more negative, reflecting the low oxygen concentration and the lower salinity compared to Anholt. Filipsson et al. (2017) showed a significant correlation between δ13CDIC and [O2] as well as with δ13CDIC and salinity in the Baltic Sea, due to the large fresh water input with negative δ13CDIC. The δ13C data for E. excavatum from Schönfeld and Numberger (2007) ranged between −1.9 ‰ and −2.3 ‰, in the same range as we measured.
5.4 Impact of seasonality on foraminiferal geochemistry
When substantial variations in water mass properties occur, not only on interannual but also on seasonal timescales (Fig. 2), it is essential to pinpoint the time of calcification of the foraminifera. This is necessary to link the geochemical signature of the foraminifera with water mass properties to eventually perform reliable downcore reconstructions. Benthic foraminifera can live up to a year or even longer (Murray, 2006; Hayward et al., 2014), resting until optimal conditions for growth and reproduction occur. Optimal conditions usually happen during spring or autumn phytoplankton blooms or during improved bottom water oxygenation (Alve, 1999; Gustafsson and Nordberg, 2001; Filipsson et al., 2004; Austin et al., 2006; Schönfeld and Numberger, 2007; Skirbekk et al., 2016). Staining foraminiferal samples with Rose Bengal at the time of collection and performing hydrographic profiling are traditionally used for direct comparison of living specimens with current water mass properties. But this approach is not optimal in a shallow or coastal setting, as there is no guarantee that the largest fraction of the calcite tests has been precipitated at the time of collection; instead the foraminifera may have calcified during a different time of the year under different salinities and temperatures. Especially in a coastal setting like the shallow Anholt site (∼30 m) where large intra- and interannual fluctuations in water mass conditions occur, it is therefore necessary to consider at what time of the year the foraminifera actually calcified.
Therefore, we performed an exercise to estimate the possible impact of such a seasonal bias on foraminiferal geochemistry. To estimate the time of calcification, we calculated δ18Osw for the studied time interval using the measured bottom water temperature and salinity data (Fig. 2) and the relationship between δ18Osw and salinity for the Baltic Sea (Fröhlich et al., 1988; Fig. 7a). Adding temperature then yielded the predicted δ18Ocalcite using the paleo-temperature equation of Epstein et al. (1953) (Fig. 7a), as modified to linear form by Bemis et al. (1998). These values were compared with the analysed average δ18Oforam values for each species for Anholt and Hanö Bay. The offset between analysed and predicted δ18O suggests that the foraminifera in Anholt calcified during spring, but that in the deeper Hanö Bay they may also have been calcifying during the time of sampling. Different conditions existed near Anholt during spring with bottom water temperature ∼2.5 ∘C lower, while salinity (+0.15) and dissolved oxygen (+0.9 mL L−1) were similar (Fig. 2). We emphasize that this approach demonstrates the importance of a possible effect of seasonal variations on foraminiferal geochemistry, possibly introducing a significant source of uncertainty.
5.5 Mn∕Caforam as proxy to reconstruct hypoxic conditions
The reconstruction of dissolved oxygen conditions in bottom water and/or pore water can be complicated because oxygen concentration and redox reactions can often rapidly change both on temporal and spatial scales. This may be one reason why foraminiferal Mn∕Ca studies (analysed using different techniques) report a large spread in Mn∕Ca values (Klinkhammer et al., 2009; Ní Fhlaithearta et al., 2010; Glock et al., 2012; Groeneveld and Filipsson, 2013; McKay et al., 2015; Koho et al., 2015, 2017; Petersen et al., 2018). Additionally, strong species-specific responses occur depending both on habitat preferences and species-specific biomineralization (e.g., Jorissen et al., 1995; Fontanier et al., 2014; Koho et al., 2017; Barras et al., 2018). Recent culturing experiments confirmed species-specific effects in A. tepida and B. marginata, which were grown at the same conditions but showed of 0.086 and 0.621, respectively (Barras et al., 2018). The Mn∕Ca data from the Kattegat indeed suggest different conditions of calcification related to habitat depths and/or seasonality for the different species. Bottom water oxygen in the Kattegat indicates oxic conditions throughout the year, but with lowest values towards the end of summer (Fig. 3; ∼3 mL L−1). Within the sediment oxygen may disappear within the top few millimetres in this region (Charrieau et al., 2018), which would be reflected in the foraminiferal Mn∕Ca (Groeneveld and Filipsson, 2013; Koho et al., 2017). Nonionellina labradorica and G. turgida are commonly referred to as deeper living infaunal species (Corliss, 1985; Jorissen et al., 1995; Schmiedl et al., 2004), which is supported by our δ13C results (Fig. 7b). Due to the lower oxygen concentrations deeper in the sediment they also show significantly higher Mn∕Ca than the shallow infaunal species (1.88 and 4.21 vs. <0.1 mmol mol−1; Table 3). Similar high values were found for G. turgida in the Gullmar Fjord (Groeneveld and Filipsson, 2013), while values in the continuously oxygenated Skagerrak stayed below 0.1 mmol mol−1. That Mn∕Ca is varying between different species depending on the preferred habitat depth at a singular location, i.e., Anholt, also suggests that the Mn∕Ca is part of the primary calcite and not deposited as diagenetic coatings. However, samples were all taken from the top 1 cm, posing the question why these deep-dwellers were present at all. Bioturbation could have brought dead specimens up into the top 1 cm. Concerning living specimens there are two options: (1) these species migrate through the sediment but only calcify when oxygen concentration is low enough (and Mn2+ thus high; Geslin et al., 2004) or (2) these species do live and calcify in the top 1 cm of the sediment but during a period of the year when bottom water or shallow sediment oxygen concentrations are low enough or when anoxic conditions occur already close to the surface (e.g., end of summer in Anholt, Fig. 2; Jorissen et al., 1995). However, our seasonality estimation for calcification of the foraminifera near Anholt was spring during which the bottom water oxygen concentration was fully oxic (5–7 mL L−1; Fig. 2a), although this does not exclude that sedimentary oxygen was already depleted close to the sediment–water interface due to the spring bloom.
The species-specific Mn∕Ca in the Kattegat indicates that a comparison with foraminiferal Mn∕Ca from Hanö Bay should ideally be made on the species-specific or at least genus level. Only the E. clavatum-selseyense complex occurs both near Anholt and in Hanö Bay. We also compare Ammonia sp. (T6) from Hanö Bay with A. falsobeccarii (Ammonia T15) from Anholt. The 25-fold increase in Mn∕Ca of the bottom water in Hanö Bay is not as clearly reflected in the foraminifera. Average Mn∕Ca in Ammonia sp. (T6) is 0.31 mmol mol−1 and average Mn∕Ca for the E. clavatum-selseyense complex is 0.88 mmol mol−1, which is 4–7 times higher than in the Kattegat (Fig. 6a). All these values are consistently lower than the deep infaunal, i.e., hypoxic, Mn∕Ca in Kattegat for N. labradorica and G. turgida (1.90 and 4.32 mmol mol−1, respectively). Possible explanations for these apparently too low foraminiferal Mn∕Ca are that (1) the bottom water oxygen concentration in Hanö Bay is low enough for the manganese to escape into the water column, (2) the manganese concentration becomes super-saturated in the pore water and precipitation of Mn carbonate occurs (e.g., rhodochrosite; Pedersen and Price, 1982; Burdige, 1993; Huckriede and Meischner, 1996), (3) the low carbonate saturation state caused dissolution lowering the Mn∕Caforam (Table 3), or (4) the foraminifera calcified during a time of the year when the oxygen concentration was higher as for example during spring 2012, and thus the Mn concentration lower (Fig. 2). Especially in the Baltic Sea, manganese is leached from anoxic sediments into hypoxic/anoxic bottom waters (Hartman, 1964; Huckriede and Meischner, 1996; Goldberg et al., 2012). This seems more likely than the precipitation of Mn carbonates, which are mainly restricted to the deepest basins in the Baltic Sea, where it is facilitated by intense sulfate reduction increasing alkalinity and promoting their precipitation (Hartman, 1964; Nehring, 1990; Huckriede and Meischner, 1996; Lenz et al., 2015).
Our results confirm that, as was also shown in previous studies, the Mn∕Ca in the foraminiferal tests does increase under hypoxic (anoxic) conditions but that the magnitude of the signal very much depends on the overall setting of their habitat (Koho et al., 2015). Species which live deeper in the sediment under hypoxic/anoxic conditions but with a kind of “cap” of oxygen on top of their habitat, either within the sediment (Kattegat) or in the bottom water (Skagerrak; Groeneveld and Filipsson, 2013) have significantly more manganese available to incorporate into their tests due to the redox cycling of the manganese (Koho et al., 2015). On the other hand, when bottom waters are already severely depleted in oxygen as in Hanö Bay (this study), significant amounts of manganese may leach into the overlying water column. This results in an elevation of foraminiferal Mn∕Ca, but not as extreme as when an “oxygen cap” is present.
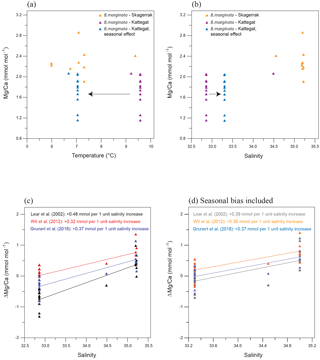
Figure 9(a) Mg∕Ca vs. temperature for B. marginata from the Anholt stations including the Skagerrak data from Groeneveld and Filipsson (2013). Blue triangles suggest the temperature of calcification when seasonality in the Kattegat is taken into account; (b) Mg∕Ca vs. salinity for B. marginata from the Anholt stations including the Skagerrak data from Groeneveld and Filipsson (2013). Blue triangles suggest the salinity of calcification when seasonality in the Kattegat is taken into account; (c) Δ(Mg∕Ca), the difference between measured and expected Mg∕Ca, for B. marginata vs. salinity for three different calibration equations; (d) Δ(Mg∕Ca), the difference between measured and expected Mg∕Ca, for B. marginata vs. salinity for three different calibration equations when possible seasonal effects as calculated in Sect. 5.3 are taken into account.
5.6 Impact of salinity on Mg∕Caforam
The comparison of Mg∕Ca with bottom water temperature is complicated in the Baltic Sea by the large variations in other water mass properties such as salinity (resulting in decreased Mg∕Casw) and carbonate chemistry between Anholt (Kattegat) and Hanö Bay, which are also affecting Mg∕Ca (e.g., Elderfield et al., 2006; Raitzsch et al., 2008; Dissard et al., 2010), and by the shallow water depth of the Anholt station such that seasonal temperature changes are relatively large (Fig. 2). The analysis of multiple species at Anholt demonstrates that species-specific comparisons and/or calibrations are needed, as there are considerable differences between species; average Mg∕Ca values vary between 0.94 mmol mol−1 for A. falsobeccarii and 1.01 mmol mol−1 for the E. clavatum-selseyense complex to as high as 1.74±0.30 mmol mol−1 for B. marginata, and 2.16 mmol mol−1 for G. turgida (Table 3).
Groeneveld and Filipsson (2013) analysed B. marginata from the Skagerrak and the Gullmar Fjord (Figs. 1, 9a, b), where average Mg∕Ca for B. marginata was 2.30 mmol mol−1. Comparing the Mg∕Ca results from the Kattegat and the Skagerrak shows that temperature is unlikely to be the dominant factor controlling foraminiferal Mg∕Ca (Fig. 9a). When a possible seasonality effect, as calculated in Sect. 5.3, is taken into account for the Anholt samples, calcification would have occurred during spring with temperatures 2.5–4 ∘C lower (and salinity 0.2–0.4 higher), similar to the Skagerrak but with lower Mg∕Ca. Bottom water salinity generally decreases from the Skagerrak to the Kattegat by ∼3 units, which is in the direction of the Mg∕Ca decrease (Fig. 9b).
To determine the possible impact of salinity on the Mg∕Ca of B. marginata, we calculated the expected Mg∕Ca (using measured bottom water temperature) based on existing calibrations including a possible seasonal bias for the Anholt location (Figs. 7, 9; Lear et al., 2002; Wit et al., 2012; Grunert et al., 2018). Due to the greater water depth of the Skagerrak location we assumed no seasonal bias there. The difference between the measured Mg∕Ca and the expected Mg∕Ca ()) shows a significant relationship (p<0.01 for each option) with salinity (Fig. 9c, d). For 1 unit increase in salinity, Mg∕Ca increases by 0.32–0.48 mmol mol−1 (r2=0.50–0.77). This influence of salinity is similar to previous studies on both planktonic foraminifera (4 % increase in Mg∕Ca per salinity unit, Globigerina bulloides; Lea et al., 1999 – 5 %, Globigerinoides ruber; Kisakürek et al., 2008) and benthic foraminifera (3.2 %–3.6 %, Ammonia tepida; Dissard et al., 2010). However, such a linear relationship would predict a Mg∕Ca of 0 at salinity of ∼26, which is unlikely since salinity in Hanö Bay was 13.7 with foraminiferal Mg∕Ca between 0.5 and 1.0 mmol mol−1 for Elphidium spp. and Ammonia sp. Despite the different species in Hanö Bay this suggests that a relationship between salinity and Mg∕Ca should be non-linear.
Salinity (and therefore also Mg∕Casw) and alkalinity are strongly correlated in seawater; this effect is particularly strong in the Baltic Sea, where the gradients are very large due to fresh water input (Ohlson and Anderson, 1991; Hjalmarsson et al., 2010). Both salinity and carbonate system parameters like alkalinity and the carbonate ion effect are known to have an impact on Mg∕Ca in open ocean settings (Elderfield et al., 2006; Raitzsch et al., 2008; Dissard et al., 2010; Huang et al., 2012; Diz et al., 2012). Accordingly, we cannot exclude that the (apparent) dependency of B. marginata Mg∕Ca on salinity, may also be caused by differences in the carbonate system parameters between the Skagerrak, Kattegat, and Baltic Sea (Table 3). Future work under controlled laboratory conditions may help to disentangle the different impacts better.
Because the species-specific Mg∕Ca for B. marginata (1.71 mmol mol−1) is higher than for Ammonia spp. (0.94 mmol mol−1) and Elphidium spp. (1.01 mmol mol−1) (Table 3), the salinity dependence for B. marginata cannot be extrapolated into Hanö Bay, where the species itself does not occur, to include this large salinity gradient (∼20 unit difference). But, when we compare the Mg∕Ca of Ammonia spp. between Anholt and Hanö Bay, the difference is minor (Anholt: 0.94±0.22 mmol mol−1; Hanö Bay: 0.82±0.13 mmol mol−1). The low sample density and relatively large scatter in the data in combination with the low-end of a possible dependency calibration prevent detection of a significant correlation. The slightly lower Mg∕Ca in Hanö Bay may also be the result of dissolution due to the low carbonate saturation state (−58.9 µmol kg−1; Table 3). Another possibility is that the sensitivity of Mg∕Ca to salinity in Ammonia spp. and also Elphidium spp. is very low or absent, as opposed to the sensitivity of Mg∕Ca to salinity in B. marginata. This was previously also concluded from two culture experiments performed on the morphospecies A. beccarii and A. tepida (salinity range 18–33; Toyofuku et al., 2011; salinity range 29.8–35.5; Diz et al., 2012), and is hinted at as predicted Mg∕Ca values when using an existing calibration are the same as the analysed values (0.96 mmol mol−1 for Anholt and 0.75 mmol mol−1 for Hanö Bay; Toyofuku et al., 2011).
5.7 Ba∕Caforam as proxy to reconstruct salinity
A strong spatial salinity gradient is one of the most prominent features of environmental change in the Baltic Sea. Proxies used to reconstruct past salinity changes include Sr isotopes on mollusks (Widerlund and Andersson, 2011; Ning et al., 2016), δ18O on rhodochrosite and mollusks (Punning et al., 1988; Huckriede and Meischner, 1996), modelling experiments (Gustafsson and Westman, 2002), and process lengths of dinoflagellate cysts (Mertens et al., 2012; Ning et al., 2017), but reconstructed absolute salinity values vary widely (Gustafsson and Westman, 2002; Ning et al., 2017; Kotthoff et al., 2017).
Absolute salinity values can be calculated from foraminiferal Ba∕Ca when the partitioning coefficient between Ba∕Ca in seawater and the foraminiferal tests is known ( (Hönisch et al., 2011; de Nooijer et al., 2017). Combining the average Ba∕Caforam of the Hanö Bay samples (both Elphidium and Ammonia) of 11.09 µmol mol−1 (n=12; σ=2.19) and the bottom water Ba∕Casw of 19.49 µmol mol−1 gives a partitioning coefficient of 0.57 (±0.02), although it cannot be excluded that Ba∕Caforam was lowered due to dissolution of the calcite. Published DBa values for cultured benthic foraminifera vary between 0.20 for A. beccarii, 0.33 for Heterostegina depressa, 0.37 for Cibicidoides wuellerstorfi, 0.5 for Cibicidoides pachyderma, and 0.78 for Amphistegina lessonii (Lea and Boyle, 1989; Havach et al., 2001; de Nooijer et al., 2017). Values of DBa determined from culturing experiments on shallow-dwelling planktonic foraminifera are lower (0.15; Lea and Spero, 1994; Hönisch et al., 2011), but DBa from deeper-dwelling planktonic foraminifera (globorotaliids) is suggested to be as high as ∼1 (Lea and Boyle, 1991). Combining the equation to determine the partitioning factor and Eq. (2) and resolving for salinity then gives:
As this equation is based on Ba∕Casw values from Hanö Bay, the equation is applicable to those areas where salinity is dominantly controlled by river runoff like the southern Baltic Sea and Baltic Proper, with little influence from the Skagerrak–North Sea. It is likely that the Ba∕Casw vs. salinity relationship changes further north in the Baltic Sea, towards the Gulf of Bothnia, as the rivers there drain through Precambrian bedrock, containing significantly less dissolved barium (Salminen, 2005).
In this study we have explored how differences in bottom water temperature, salinity, and dissolved oxygen between the Kattegat and Hanö Bay (the Baltic Proper) affect benthic foraminiferal assemblages (identified morphologically and molecularly), and the test geochemistry of several species of benthic foraminifera.
We show that metal ∕ Ca ratios in the water column are directly related to the water mass conditions. Mn∕Casw is determined by the oxygen content of the water that determines the redox state of manganese. Mn∕Casw is low in the Kattegat where the water is oxic, but increases a 100-fold in the deeper, hypoxic water at Hanö Bay. Mg∕Casw in the brackish Hanö Bay is lower than in the marine Kattegat due to the large fresh water input. Ba∕Casw in the Baltic Proper is dominantly controlled by the large fresh water input increasing when salinity decreases.
Most of the foraminifera found at the Anholt site (Kattegat) are representative of shelf species typically found in the northeast Atlantic, although with a lower biodiversity: A. batava (Ammonia T3S), A. falsobeccarii (Ammonia T15), B. marginata, C. laevigata, and N. labradorica, which are intolerant of the low salinities in the Baltic Proper. On the other hand, Ammonia sp. (T6) and Elphidium albiumbilicatum (Elphidium S7) were only identified in Hanö Bay and Arkona Basin, showing their preference for brackish water. Elphidium clavatum (Elphidium S4) was identified at all three sites, being much less sensitive to salinity variations (euryhaline) than the other species recorded in this study. Therefore, salinity appears to be the most important controlling factor for benthic foraminiferal distribution in the studied area, although hypoxia may play a role too.
This is the first study on benthic foraminiferal geochemistry to combine traditional morphological identification and molecular characterization of species. Molecular identification fully resolves the Ammonia and Elphidium taxa at species level, enabling the identification of their specific ecological preferences in order to develop their potential as paleoenvironmental proxies with greater precision. Since Ammonia sp. (T6) and A. batava-A. falsobeccarii (T3S and T15) can be easily morphologically distinguished from one another (cf. tepida and beccarii morphotypes), and inhabit different environments, they show the greatest potential for paleoenvironmental studies.
Foraminiferal Mn∕Ca, Mg∕Ca, and Ba∕Ca mostly reflect the differences seen in the bottom water characteristics between Kattegat and Baltic Proper. Mn∕Ca ratios in Elphidium spp. and Ammonia spp. are higher in the Baltic Proper than the Kattegat which reflects the lower oxygen conditions there. Since Mn2+ can escape into the hypoxic bottom water, the Mn∕Caforam does not record values as high as when the Mn2+ remains available in the pore water. In the Kattegat, Mn∕Ca of species which live deeper in the sediment (N. labradorica and G. turgida) have much higher Mn∕Ca because the oxic bottom water conditions prevent Mn2+ from escaping into the water column.
Mg∕Ca shows a dependence on salinity in B. marginata, while Mg∕Ca in Elphidium spp. and Ammonia spp. does not seem to be affected. However, as salinity and alkalinity are highly correlated, it is not possible to separate these two factors. Ba∕Caforam in the Baltic Proper reflects changes in salinity, but this does not apply for the Kattegat samples, as the salinity changes in the Kattegat are mainly controlled by inflow of fully marine water from the Skagerrak–North Sea. The resulting dependency of Ba∕Caforam on salinity for Elphidium spp. and Ammonia spp. shows a decrease of 1.12 µmol mol−1 for each unit increase in salinity.
Our data demonstrate that the foraminiferal assemblages in the Baltic Sea are strongly dependent on the prevailing water mass conditions. Abundance and diversity of species decrease when salinity decreases. The new calibrations between water mass properties, seawater element ∕ Ca ratios, and foraminiferal element ∕ Ca ratios generated within this study can be used on downcore benthic foraminiferal records from the Baltic Sea to regionally reconstruct how bottom water temperature, salinity, and oxygen concentrations changed in the past.
All geochemical data generated in this paper are reported either in the text as tables or in the Supplement. Newly generated genetic sequences were deposited in the GenBank database (accession numbers MF170098–MF170141).
The supplement related to this article is available online at: https://doi.org/10.5194/jm-37-403-2018-supplement.
JG, HLF, WENA, and KD designed and interpreted the study. HLF, JG, WENA, and DM performed the field work. JG performed the geochemical analyses; HLF, WENA, and DMC did the faunal analyses; KD, CB, and MS performed the genetic analyses. All authors contributed to analysing the data and writing the paper.
The authors declare that they have no conflict of interest.
We thank the captain and the crew of the R/V Skagerak, Silvana Pape,
Henning Kuhnert, and Lisa Schönborn for laboratory assistance; Kath Evans for her
contribution towards the molecular project; Karen-Luise Knudsen and Laurie Charrieau
for discussing foraminifer identification issues; Kirsty Edgar
(editor), Manuel Weinkauf and an anonymous reviewer for their constructive
comments. We acknowledge funding through the Swedish Research Council (VR)
(project no. 621-2011-5090), the German Research Council (project GR
3528/3-1), the Lamm Foundation, the Centre for Environmental and Climate
Research at Lund University for Jeroen Groeneveld's guest research stay, NERC grants
NE4/G018502/1 and NE/G020310/1 to William E. N. Austin, and the University of Bremen for
covering the article processing costs for this open-access publication. The
hydrographic data used in the project is collected from SMHI's database
SHARK. The SHARK data collection is organized by the environmental
monitoring programme and funded by the Swedish Environmental Protection
Agency.
The article processing charges for this open-access
publication were covered by the University of Bremen.
Edited by: Kirsty Edgar
Reviewed by: Manuel Weinkauf and one anonymous referee
Alve, E.: Colonization of new habitats by benthic foraminifera: A review, Earth Sci. Rev., 46, 167–185, 1999.
Alve, E. and Murray, J. W.: Marginal marine environments of the Skagerrak and Kattegat: a baseline study of living (stained) benthic foraminiferal ecology, Palaeogeogr. Palaeocl., 146, 171–193, 1999.
Andrén, T., Jørgensen, B. B., Cotterill, C., and Expedition 347 Scientists: Baltic Sea Paleoenvironment, Proc. IODP, 347, College Station, TX (Integrated Ocean Drilling Program), https://doi.org/10.2204/iodp.proc.347.2015, 2015.
Anjar, J., Adrielsson, L., Bennike, O., Björck, S., Filipsson, H. L., Groeneveld, J., Knudsen, K. L., Larsen, N. K., and Möller, P.: Palaeoenvironmental history of the Baltic Sea basin during Marine Isotope Stage 3, Quaternary Sci. Rev., 34, 81–92, 2012.
Austin, W. E. N., Cage, A. G., and Scourse, J. D.: Mid-latitude shelf seas: a NW European perspective on the seasonal dynamics of temperature, salinity, and oxygen isotopes, Holocene, 16, 937–947, 2006.
Bahr, A., Schönfeld, J., Hofmann, J., Voigt, S., Aurahs, R., Kucera, M., Flögel, S., Jentzen, A., and Gerdes, A.: Comparison of Ba∕Ca and δ18Owater as freshwater proxies: A multi-species core-top study on planktonic foraminifera from the vicinity of the Orinoco River mouth, Earth Planet. Sc. Lett., 383, 45–57, 2013.
Barker, S., Greaves, M., and Elderfield, H.: A study of cleaning procedures used for foraminiferal Mg∕Ca paleothermometry, Geochem. Geophys. Geosys., 4, 8407, https://doi.org/10.1029/2003GC000559, 2003.
Barras, C., Mouret, A., Nardelli, M. P., Metzger, E., Petersen, J., La, C., Filipsson, H. L., and Jorissen, F.: Experimental calibration of manganese incorporation in foraminiferal calcite, Geochim. Cosmochim. Ac., 237, 49–64, 2018.
Barrientos, N., Lear, C. H., Jakobsson, M., Stranne, C., O'Regan, M., Cronin, T. M., Gukov, A. Y., and Coxall, H. K.: Arctic Ocean benthic foraminifera Mg∕Ca ratios and global Mg∕Ca-temperature calibrations: New constraints at low temperatures, Geochim. Cosmochim. Ac., 236, 240–259, https://doi.org/10.1016/j.gca.2018.02.036, 2018.
Bauch, H. A., Erlenkeuser, H., Bauch, D., Mueller-Lupp, T., and Taldenkova, E.: Stable oxygen and carbon isotopes in modern benthic foraminifera from the Laptev Sea shelf: implications for reconstructing proglacial and profluvial environments in the Arctic, Mar. Micropaleontol., 51, 285–300, 2004.
Bemis, B. E., Spero, H. J., Bijma, J., and Lea, D. W.: Reevaluation of the oxygen isotopic composition of planktonic foraminifera: Experimental results and revised paleotemperature equations, Paleoceanography, 13, 150–160, 1998.
Bernard, P. C., van Grieken, R. E., and Brügmann, L.: Geochemistry of suspended matter from the Baltic Sea. 1. Results of individual particle characterization by automated electron microprobe, Mar. Chem., 26, 155–177, 1989.
Björck, S.: A review of the history of the Baltic Sea, 13.0–8.0 ka BP, Quat. Internat., 27, 19–40, 1995.
Björck, S.: The late quaternary development of the Baltic Sea Basin, in: Assessment of Climate Change for the Baltic Sea Basin, edited by: The BACC Author Team, Springer-Verlag, Berlin-Heidelberg, 398–407, 2008.
Boström, K., Wiborg, L., and Ingri, J.: Geochemistry and origin of ferromanganese concretions in the Gulf of Bothnia, Mar. Geol., 50, 1–24, 1982.
Branson, O., Redfern, S. A. T., Tyliszczak, T., Sadekov, A., Langer, G., Kimoto, K., and Elderfield, H.: The coordination of Mg in foraminiferal calcite, Earth Planet. Sc. Lett., 383, 134–141, 2013.
Burdige, D. J.: The biogeochemistry of manganese and iron reduction in marine sediments, Earth Sci. Rev., 35, 249–284, 1993.
Burton, J. D., Statham, P. J., and Elderfield, H.: Trace metals as tracers in the ocean, Philos. T. R. Soc. Lond., 325, 127–145, 1988.
Butruille, C., Krossa, V. R., Schwab, C., and Weinelt, M.: Reconstruction of mid- to late-Holocene winter temperatures in the Skagerrak region using benthic foraminiferal Mg∕Ca and δ18O, Holocene, 27, 1–10, https://doi.org/10.1177/0959683616652701, 2016.
Calvert, S. E. and Pedersen, T. F.: Geochemistry of recent oxic and anoxic marine sediments: Implications for the geological record, Mar. Geol., 113, 67–88, 1993.
Canfield, D. E., Thamdrup, B., and Hansen, J. W.: The anaerobic degradation of organic matter in Danish coastal sediments: Iron reduction, manganese reduction, and sulfate reduction, Geochim. Cosmochim. Ac., 57, 3867–3883, 1993.
Charrieau, L. M., Filipsson, H. L., Ljung, K., Chierici, M., Knudsen, K. L., and Kritzberg, E.: The effects of multiple stressors on the distribution of coastal benthic foraminifera: A case study from the Skagerrak-Baltic Sea region, Mar. Micropaleontol., 139, 42–56, 2018.
Conley, D. J., Carstensen, J., Aigars, J., Axe, P., Bonsdorff, E., Eremina, T., Haahti, B.-M., Humborg, C., Jonsson, P., Kotta, J., Lännegren, C., Larsson, U., Maximov, A., Medina, M. R., Lysiak-Pastuszak, E., Remeikaite-Nikiene, N., Walve, J., Wilhelms, S., and Zillén, L.: Hypoxia is increasing in the coastal zone of the Baltic Sea, Environ. Sci. Technol., 45, 6777–6783, 2011.
Conradsen, K., Bergsten, H., Knudsen, K. L., Nordberg, K., and Seidenkrantz, M. S.: Recent benthic foraminiferal distribution in the Kattegat-Skagerrak, Scandinavia, J. Foramin. Res., 32, 53–68, 1994.
Corliss, B. H.: Microhabitats of benthic foraminifera within deep-sea sediments, Nature, 314, 435–438, 1985.
Darling, K. F., Schweizer, M., Knudsen, K. L., Evans, K. M., Bird, C., Roberts, A., Filipsson, H. L., Kim, J.-H., Gudmundsson, G., Wade, C. M., Sayer, M. D., and Austin, W. E. N.: The genetic diversity, phylogeography and morphology of Elphidiidae (foraminifera) in the Northeast Atlantic, Mar. Micropaleontol., 129, 1–23, https://doi.org/10.1016/j.marmicro.2016.09.001, 2016.
Dekens, P. S., Lea, D. W., Pak, D. K., and Spero, H. J.: Core top calibration of Mg∕Ca in tropical foraminifera: Refining paleotemperature estimation, Geochem., Geophys,. Geosys., 3, 1–29, https://doi.org/10.1029/2001GC000200, 2002.
de Nooijer, L. J., Brombacher, A., Mewes, A., Langer, G., Nehrke, G., Bijma, J., and Reichart, G.-J.: Ba incorporation in benthic foraminifera, Biogeosciences, 14, 3387–3400, https://doi.org/10.5194/bg-14-3387-2017, 2017.
Diaz, R. J. and Rosenberg, R.: Spreading dead zones and consequences for marine ecosystems, Science, 321, 926–929, https://doi.org/10.1126/science.1156401, 2008.
Dijkstra, N., Quintana Krupinski, N. B., Yamane, M., Obrochta, S. P., Yokoyama, Y., and Slomp, C. P.: Holocene refreshing and reoxygenation of a Bothnian Sea estuary led to enhanced phosphorus burial, Estuar. Coast., 41, 139–157, 2018.
Dissard, D., Nehrke, G., Reichart, G. J., and Bijma, J.: The impact of salinity on the Mg∕Ca and Sr∕Ca ratio in the benthic foraminifera Ammonia tepida: Results from culture experiments, Geochim. Cosmochim. Ac., 74, 928–940, 2010.
Diz, P., Barras, C., Geslin, E., Reichart, G. J., Metzger, E., Jorissen, F., and Bijma, J.: Incorporation of Mg and Sr and oxygen and carbon stable isotope fractionation in cultured Ammonia tepida, Mar. Micropaleontol., 92–93, 16–28, 2012.
Dyrssen, D.: The Baltic-Kattegat-Skagerrak estuarine system, Estuaries, 16, 446–452, 1993.
Elderfield, H. and Ganssen, G.: Past temperatures and δ18O of surface ocean waters inferred from foraminiferal Mg∕Ca ratios, Nature, 405, 442–445, 2000.
Elderfield, H., Yu. J., Anand, P., Kiefer, T., and Nyland, B.: Calibrations for benthic foraminiferal Mg∕Ca paleothermometry and the carbonate ion hypothesis, Earth Planet. Sc. Lett., 250, 633–649, https://doi.org/10.1016/j.epsl.2006.07.041, 2006.
Ellis, B. F. and Messina, A. R.: Catalogue of Foraminifera, Micropaleontology Press, New York, The American Museum of Natural History, 1940.
Epstein, S., Buchsbaum, R., Lowenstam, H. A., and Urey, H. C.: Revised carbonate-water isotopic temperature scale, Geol. Soc. Am. Bull., 64, 1315–1325, 1953.
Erbs-Hansen, D. R., Knudsen, K. L., Gary, A. C., Gyllencreutz, R., and Jansen, E.: Holocene climatic development in Skagerrak, eastern North Atlantic: Foraminiferal and stable isotopic evidence, Holocene, 22, 301–312, 2012.
Evans, D. and Müller, W.: Deep time foraminifera Mg∕Ca paleothermometry: Nonlinear correction for secular change in seawater Mg∕Ca, Paleoceanography, 27, PA4205, https://doi.org/10.1029/2012PA002315, 2012.
Feyling-Hanssen, R. W.: Foraminifera in Late Quaternary Deposits from the Oslofjord Area, Skrifter (Norges geologiske undersøkelse), Universitetsforlaget, Oslo, 225, 1964.
Feyling-Hanssen, R. W.: The foraminifer Elphidium excavatum (Terquem) and its variant forms, Micropaleontology, 18, 337–354, 1972.
Feyling-Hanssen, R. W., Jørgensen, J. A., Knudsen, K. L., and Andersen, A. I.: Late Quaternary foraminifera from Vendsyssel, Denmark and Sandnes, Norway, Bull. Geol. Soc. Den., 21, 61–317, 1971.
Filipsson, H. L. and Nordberg, K.: Climate variations, an overlooked factor influencing the recent marine environment. An example from Gullmar Fjord, Sweden, illustrated by benthic foraminifera and hydrographic data, Estuaries, 27, 867–881, 2004a.
Filipsson, H. L. and Nordberg, K.: A 200-year environmental record of a low-oxygen fjord, Sweden, elucidated by benthic foraminifera, sediment characteristics and hydrographic data, J. Foram. Res., 34, 277–293, https://doi.org/10.2113/34.4.277, 2004b.
Filipsson, H. L., Nordberg, K., and Gustafsson, M.: Seasonal study of δ18O and δ13C in living (stained) benthic foraminifera from two Swedish fjords, Mar. Micropaleontol., 53, 159–172, 2004.
Filipsson, H. L., McCorkle, D. C., Mackensen, A., Bernhard, J. M., Andersson, L. S., Naustvoll, L. J., Caballero-Alfonso, A. M., Nordberg, K., and Danielssen, D. S.: Seasonal variability of stable carbon isotopes (δ13CDIC) in the Skagerrak and the Baltic Sea: Distinguishing between mixing and biological productivity, Palaeogeogr. Palaeocl., 483, 15–30, 2017.
Fontanier, C., Duros, P., Toyofuku, T., Oguri, K., Koho, K. A., Buscail, R., Gremare, A., Radakovitch, O., Deflandre, B., De Nooijer, L. J., Bichon, S., Goubet, S., Ivanovsky, A., Chabaud, G., Menniti, C., Reichart, G.-J., and Kitazato, H.: Living (stained) deep-sea foraminifera off Hachinohe (NE Japan, Western Pacific): Environmental interplay in oxygen-depleted ecosystems, J. Foram. Res., 44, 281–299, 2014.
Froelich, P. N., Klinkhammer, G. P., Bender, M. L., Luedtke, N. A., Heath, G. R., Cullen, D., Dauphin, P., Hammond, D., Hartman, B., and Maynard, V.: Early oxidation of organic matter in pelagic sediments of the eastern equatorial Atlantic: suboxic diagenesis, Geochim. Cosmochim. Ac., 43, 1075–1090, 1979.
Fröhlich, K., Grabczak, J., and Rozanski, K.: Deuterium and oxygen-18 in the Baltic Sea, Chem. Geol., 72, 77–83, 1988.
Geslin, E., Heinz, P., Jorissen, F., and Hemleben, Ch.: Migratory responses of deep-sea benthic foraminifera to variable oxygen conditions: laboratory investigations, Mar. Micropaleontol., 53, 227–243, 2004.
Glock, N., Eisenhauer, A., Liebetrau, V., Wiedenbeck, M., Hensen, C., and Nehrke, G.: EMP and SIMS studies on Mn∕Ca and Fe∕Ca systematics in benthic foraminifera from the Peruvian OMZ: a contribution to the identification of potential redox proxies and the impact of cleaning protocols, Biogeosciences, 9, 341–359, https://doi.org/10.5194/bg-9-341-2012, 2012.
Goldberg, T., Archer, C., Vance, D., Thamdrup, B., McAnena, A., and Poulton, S. W.: Controls on Mo isotope fractionation in a Mn-rich anoxic marine sediment, Gullmar Fjord, Sweden, Chem. Geol., 296–297, 73–82, 2012.
Grasshoff, K. and Voipio, A.: Chemical Oceanography, in: The Baltic Sea, edited by: Voipio, A., Elsevier Oceanography Series 30, Amsterdam, 183–218, 1981.
Greaves, M., Caillon, N., Rebaubier, H., Bartoli, G., Bohaty, S., Cacho, I., Clarke, L., Cooper, M. Daunt, C., Delaney, M., deMenocal, P., Dutton, A., Eggins, S., Elderfield, H., Garbe-Schönberg, D., Goddard, E., Green, D., Groeneveld, J., Hastings, D., Hathorne, E., Kimoto, K., Klinkhammer, G., Labeyrie, L., Lea, D. W., Marchitto, T., Martinez-Boti, M. A., Mortyn, P. G., Ni, Y., Nürnberg, D., Paradis, G., Pena, L., Quinn, T., Rosenthal, Y., Russell, A., Sagawa, T., Sosdian, S., Stott, L., Tachikawa, K., Tappa, E., Thunell, R., and Wilson, P. A.: Interlaboratory comparison study of calibration standards for foraminiferal Mg∕Ca thermometry, Geochem. Geophys. Geosyst., 9, Q08010, https://doi.org/10.1029/2008GC001974, 2008.
Groeneveld, J. and Filipsson, H. L.: Mg∕Ca and Mn∕Ca ratios in benthic foraminifera: the potential to reconstruct past variations in temperature and hypoxia in shelf regions, Biogeosciences, 10, 5125–5138, https://doi.org/10.5194/bg-10-5125-2013, 2013.
Grunert, P., Rosenthal, Y., Jorissen, F., Holbourn, A., Zhou, X., and Piller, W.: Mg∕Ca-temperature calibration for costate Bulimina species (B. costata, B. inflata, B. mexicana): A paleothermometer for hypoxic environments, Geochim. Cosmochim. Ac., 220, 36–54, 2018.
Gustafsson, M. and Nordberg, K.: Living (stained) benthic foraminifera and their response to the seasonal hydrographic cycle, periodic hypoxia and to primary production in Havstens Fjord on the Swedish west coast, Estuar. Coast. Shelf Sci., 51, 743–761, 2000.
Gustafsson, M. and Nordberg, K., Living (stained) benthic foraminiferal response to primary production and hydrography in the deepest part of the Gullmar Fjord, Swedish west coast, with comparisons to Höglund's 1927 material, J. Foramin. Res., 31, 2–11, 2001.
Gustafsson, B. G. and Westman, P.: On the causes for salinity variations in the Baltic Sea during the last 8500 years, Paleoceanography, 17, 1–14, https://doi.org/10.1029/2000PA000572, 2002.
Hall, J. M. and Chan, L. -H.: Li/Ca in multiple species of benthic and planktonic foraminifera: Thermocline, latitudinal, and glacial-interglacial variation, Geochim. Cosmochim. Ac., 68, 529–545, 2004.
Hall, T. A.: BioEdit: a user-friendly biological sequence alignment editor and analysis program for Windows 95/98/NT, Nucl. Acid. S., 41, 95–98, 1999.
Hammer, Ø., Harper, D. A. T., and Ryan, P. D.: PAST: Paleontological Statistics Software Package for Education and Data Analysis, Palaeontol. Electron., 4, 4, 2001.
Hartman, M.: Zur Geochemie von Mangan und Eisen in der Ostsee, Meyniana, 14, 3–20, 1964.
Hathorne, E. C., James, R. H., and Lampitt, R. S.: Environmental versus biomineralization controls on the intratest variation in the trace element composition of the planktonic foraminifera G. inflata and G. scitula, Paleoceanography, 24, PA4204, https://doi.org/10.1029/2009PA001742, 2009.
Havach, S. M., Chandler, G. T., Wilson-Fenelli, A., and Shaw, T. J.: Experimental determination of trace element partition coefficients in cultured benthic foraminifera, Geochim. Cosmochim. Ac., 65, 1277–1283, 2001.
Haynes, J. R.: Supposed pronounced ecophenotypy in foraminifera, J. Micropaleontology, 11, 59–63, 1992.
Hayward, B. W., Holzmann, M., Grenfell, H. R., Pawlowski, J., and Triggs, C. M.: Morphological distinction of molecular types in Ammonia – towards a taxonomic revision of the world's most commonly misidentified foraminifera, Mar. Micropaleontol., 50, 237–271, 2004.
Hayward, B. W., Figueira, B. O., Sabaa, A. T., and Buzas, M. A.: Multi-year life spans of high salt marsh agglutinated foraminifera from New Zealand, Mar. Micropaleontol., 109, 54–65, 2014.
Hjalmarsson, S., Chierici, M., and Anderson, L. G.: Carbon dynamics in a productive coastal region – The Skagerrak, J. Mar. Sys., 82, 245–251, 2010.
Holzmann, M. and Pawlowski, J.: Taxonomic relationships in the genus Ammonia (Foraminifera) based on ribosomal DNA sequences, J. Micropaleont., 19, 85–95, 2000.
Holzmann, M. and Pawlowski, J.: An updated classification of rotaliid foraminifera based on ribosomal DNA phylogeny, Mar. Micropaleontol., 132, 18–34, 2017.
Hönisch, B., Allen, K. A., Russell, A. D., Eggins, S. M., Bijma, J., Spero, H. J., Lea, D. W., and Yu, J.: Planktic foraminifers as recorders of sea water Ba∕Ca, Mar. Micropaleontol., 79, 52–57, 2011.
Huang, E., Mulitza, S., Paul, A., Groeneveld, J., Steinke, S., and Schulz, M.: Response of eastern tropical Atlantic central waters to Atlantic meridional overturning circulation changes during the Last Glacial Maximum and Heinrich Stadial 1, Paleoceanography, 27, PA3229, https://doi.org/10.1029/2012PA002294, 2012.
Huckriede, H. and Meischner, D.: Origin and environment of manganese-rich sediments within black-shale basins, Geochim. Cosmochim. Ac., 60, 1399–1413, 1996.
Ingri, J., Löfvendahl, R., and Boström, K.: Chemistry of suspended particles in the southern Baltic Sea, Mar. Chem., 32, 73–87, 1991.
Jakobsson, M., Björck, S., Alm, G., Andrén, T., Lindeberg, G., and Svensson, N. O.: Reconstructing the Younger Dryas ice dammed lake in the Baltic Basin: bathymetry, area and volume, Global Planet. Change, 57, 355–370, 2007.
Jilbert, T. and Slomp, C. P.: Rapid high-amplitude variability in Baltic Sea hypoxia during the Holocene, Geology, 41, 1183–1186, 2013.
Jones, R. W.: Henry Bowman Brady (1835–1891): the man, the scientist and the scientific legacy, in: Landmarks in Foraminiferal Micropalaeontology, History and Development, edited by: Bowden, J., Gregory, F. J., and Henderson, A. S., The Micropalaentological Society, Special Publications, Geological Society, London, 23–30, 2013.
Jorissen, F. J., de Stigter, H. C., and Widmark, J. G. V.: A conceptual model explaining benthic foraminiferal microhabitats, Mar. Micropaleontol., 26, 3–15, 1995.
Keeling, R. F. and Garcia, H. E.: The change in oceanic O2 inventory associated with recent global warming, P. Natl. Acad. Sci. USA, 99, 7848–7853, 2002.
Kisakürek, B., Eisenhauer, T., Böhm, F., Garbe-Schönberg, D., and Erez, J.: Controls on shell Mg∕Ca and Sr∕Ca in cultured planktonic foraminifera, Globigerinoides ruber (white), Earth Planet. Sc. Lett., 273, 260–269, https://doi.org/10.1016/j.epsl.2008.06.026, 2008.
Klinkhammer, G. P., Mix, A. C., and Haley, B. A.: Increased dissolved terrestrial input to the coastal ocean during the deglaciation, Geochem. Geophys. Geosys., 10, Q03009, https://doi.org/10.1029/2008GC002219, 2009.
Koho, K. A., de Nooijer, L. J., and Reichart, G. J.: Combining benthic foraminiferal ecology and shell Mn∕Ca to deconvolve past bottom water oxygenation and paleoproductivity, Geochim. Cosmochim. Ac., 165, 294–306, 2015.
Koho, K. A., de Nooijer, L. J., Fontanier, C., Toyofuku, T., Oguri, K., Kitazato, H., and Reichart, G.-J.: Benthic foraminiferal Mn∕Ca ratios reflect microhabitat preferences, Biogeosciences, 14, 3067–3082, https://doi.org/10.5194/bg-14-3067-2017, 2017.
Kotilainen, A. T., Arppe, L., Dobosz, S., Jansen, E., Kabel, K., Karhu, J., Kotilainen, M. M., Kuijpers, A., Lougheed, B. C., Meier, H. E. M., Moros, M., Neumann, T., Porsche, C., Poulsen, N. Rasmussen, P., Ribeiro, S., Risebrobakken, B., Ryabchuk, D., Schimanke, S., Snowball, I., Spiridonov, M., Virtasalo, J. J., Weckström, K., Witkowski, A., and Zhamoida, V.: Echoes from the past: A healthy Baltic Sea requires more effort, Ambio, 43, 60–68, 2014.
Kotthoff, U., Groeneveld, J., Ash, J. L., Fanget, A.-S., Krupinski, N. Q., Peyron, O., Stepanova, A., Warnock, J., Van Helmond, N. A. G. M., Passey, B. H., Clausen, O. R., Bennike, O., Andrén, E., Granoszewski, W., Andrén, T., Filipsson, H. L., Seidenkrantz, M.-S., Slomp, C. P., and Bauersachs, T.: Reconstructing Holocene temperature and salinity variations in the western Baltic Sea region: a multi-proxy comparison from the Little Belt (IODP Expedition 347, Site M0059), Biogeosciences, 14, 5607–5632, https://doi.org/10.5194/bg-14-5607-2017, 2017.
Kremling, K. and Wilhelm, G.: Recent increase of the calcium concentrations in the Baltic Sea waters, Mar. Poll. Bull., 34, 763–767, 1997.
Kristensen, P. H. and Knudsen, K. L.: Palaeoenvironments of a complete Eemian sequences at Mommark, South Denmark: foraminifera, ostracods and stable isotopes, Boreas, 35, 349–366, 2006.
Kristjánsdóttir, G. B., Lea, D. W., Jennings, A. E., Pak, D. K., and Belanger, C.: New spatial Mg∕Ca-temperature calibrations for three Arctic, benthic foraminifera and reconstruciton of north Iceland shelf temperature for the past 4000 years, Geochem. Geophys. Geosys., 8, Q03P21, https://doi.org/10.1029/2006GC001425, 2007.
Lea, D. and Boyle, E.: Barium content of benthic foraminifera controlled by bottom-water composition, Nature, 338, 751–753, 1989.
Lea, D. W. and Boyle, E. A.: Barium in planktonic foraminifera, Geochim. Cosmochim. Ac., 55, 3321–3331, 1991.
Lea, D. W. and Spero, H. J.: Assessing the reliability of paleochemical tracers: Barium uptake in the shells of planktonic foraminifera, Paleoceanography, 9, 445–452, 1994.
Lea, D. W., Mashiotta, T. A., and Spero, H. J.: Controls on magnesium and strontium uptake in planktonic foraminifera determined by live culturing, Geochim. Cosmochim. Ac., 63, 2369–2379, 1999.
Lear, C. H., Rosenthal, Y., and Slowey, N.: Benthic foraminiferal Mg∕Ca-paleothermometry: A revised core-top calibration, Geochim. Cosmochim. Ac., 66, 3375–3387, 2002.
Lenz, C., Jilbert, T., Conley, D. J., and Slomp, C. P.: Hypoxia-driven variations in iron and manganese shuttling in the Baltic Sea over the past 8 kyrs, Geochem. Geophys. Geosys., 16, 3754–3766, https://doi.org/10.1002/2015GC005960, 2015.
Loeblich, A. R. and Tappan, H.: Part C, Protista 2, Sarcodina, Chiefly `Thecamoebians' and Foraminiferida, in: Treatise on Invertebrate Paleontology, edited by: Moore, R. C., The Geological Society of America and the University of Kansas, 900 pp., 1964.
Löfvendahl, R., Åberg, G., and Hamilton, P. J.: Strontium in rivers of the Baltic Basin, Aquat. Sci., 52, 315–329, 1990.
Lutze, G. F.: Zur Foraminiferen-Fauna der Ostsee, Meyniana, 15, 75–142, 1965.
Madden, T.: The BLAST Sequence Analysis Tool, in: The NCBI Handbook, edited by: McEntyre, J. and Ostell, J., Bethesda (MD): National Center for Biotechnology Information (US), Chapter 16, available at: http://www.ncbi.nlm.nih.gov/books/NBK21097/ (last access: June 2016), 2002.
Matthäus, W. and Schinke, H.: The influence of river runoff on deep water conditions of the Baltic Sea, Hydrobiologia, 393, 1–10, 1999.
McCorkle, D. C., Corliss, B. H., and Farnham, C. A.: Vertical distributions and stable isotopic compositions of live (stained) benthic foraminifera from the North Carolina and California continental margins, Deep-Sea Res. Pt. I, 44, 983–1024, 1997.
McKay, C. L., Groeneveld, J., Filipsson, H. L., Gallego-Torres, D., Whitehouse, M. J., Toyofuku, T., and Romero, O. E.: A comparison of benthic foraminiferal Mn∕Ca and sedimentary Mn∕Al as proxies of relative bottom-water oxygenation in the low-latitude NE Atlantic upwelling system, Biogeosciences, 12, 5415–5428, https://doi.org/10.5194/bg-12-5415-2015, 2015.
McKenna, C., Berx, B., and Austin, W. E. N.: The decomposition of the Faroe-Shetland Channel water masses using Parametric Optimum Multi-Parameter analysis, Deep-Sea Res. Pt. I, 107, 9–21, 2016.
Mehrbach, C., Culberso, C. H., Hawley, J. E., and Pytkowic, R. M.: Measurement of apparent dissociation constants of carbonic acid in seawater at atmospheric pressure, Limnol. Oceanogr., 18, 897–907, 1973.
Meier, H. E. M., Andersson, H. C., Eilola, K., Gustafsson, B. G., Kuznetsov, I., Müller-Karulis, B., Neumann, T., and Savchuk, O. P.: Hypoxia in future climates: A model ensemble study for the Baltic Sea, Geophys. Res. Lett., 38, L24608, https://doi.org/10.1029/2011GL049929, 2011.
Mertens, K. N., Bringue, M., van Nieuwenhove, N., Takano, Y., Pospelova, V., Rochon, A., de Vernal, A., Radi, T., Dale, B., Patterson, R. T., Weckström, K., Andren, E., Louwye, S., and Matsuoka, K.: Process length variation of the cyst of the dinoflagellate Protoceratium reticulatum in the North Pacific and Baltic-Skagerrak region: calibration as an annual density proxy and first evidence of pseudo-cryptic speciation, J. Quaternary Sci., 27, 734–744, 2012.
Mohrholz, V., Naumann, M., Nausch, G., Krüger, S., and Gräwe, U.: Fresh oxygen for the Baltic Sea – An exceptional saline inflow after a decade of stagnation, J. Mar. Sys., 148, 152–166, 2015.
Murray, J. W. (Ed.): Ecology and applications of benthic foraminifera, Cambridge University Press, Cambridge, 426 pp., 2006.
Murray, J. W.: Biodiversity of living benthic foraminifera: How many species are there?, Mar. Micropaleontol., 64, 163–176, https://doi.org/10.1016/j.marmicro.2007.04.002, 2007.
Murray, J. W. and Alve, E.: The distribution of agglutinated Foraminifera in NW European seas: baseline data for the interpretation of fossil assemblages, Palaeontol. Electron., 14, 14A, 1–41, 2011.
Nardelli, M. P., Barras, C., Metzger, E., Mouret, A., Filipsson, H. L., Jorissen, F., and Geslin, E.: Experimental evidence for foraminiferal calcification under anoxia, Biogeosciences, 11, 4029–4038, https://doi.org/10.5194/bg-11-4029-2014, 2014.
Nehring, D.: Hydrographic and chemical conditions in the Western and Central Baltic Sea from 1979 to 1988 – a comparison, Mar. Sci. Rep., 2, 2–45, 1990.
Ní Fhlaithearta, S., Reichart, G.-J., Jorissen, F. J., Fontanier, C., Rohling, E. J., Thomson, J., and De Lange, G. J.: Reconstructing the seafloor environment during sapropel formation using benthic foraminiferal trace metals, stable isotopes, and sediment composition, Paleoceanography, 25, PA4225, https://doi.org/10.1029/2009PA001869, 2010.
Ning, W., Ghosh, A., Jilbert, T., Slomp, C. P., Khan, M., Nyberg, J., Conley, D. J., and Filipsson, H. L.: Evolving coastal character of a Baltic Sea inlet during the Holocene shoreline regression: impact on coastal zone hypoxia, J. Paleolimno., 55, 319–338, https://doi.org/10.1007/s10933-016-9882-6, 2016.
Ning, W., Andersson, P. S., Ghosh, A., Khan, M., and Filipsson, H. L.: Quantitative salinity reconstructions of the Baltic Sea during the mid-Holocene, Boreas, 46, 100–110, https://doi.org/10.1111/bor.12156, 2017.
Nordberg, K. and Bergsten, H.: Biostratigraphic and sedimentological evidence of hydrographic changes in the Kattegat during the later part of the Holocene, Mar. Geol., 83, 135–158, 1988.
Nürnberg, D.: Taking the temperature of past ocean surfaces, Science, 289, 1698–1699, https://doi.org/10.1126/science.289.5485.1698, 2000.
Ohlson, M. and Anderson, L.: Recent investigation of total carbonate in the Baltic Sea: changes from the past as a result of acid rain?, Mar. Chem., 30, 259–267, 1991.
Osterman, L. E., Poore, R. Z., Swarzenski, P. W., and Turner, R. E.: Reconstructing a 180 yr record of natural and anthropogenic induced low-oxygen conditions from Louisiana continental shelf sediments, Geology, 33, 329–332, https://doi.org/10.1130/G21341.1, 2005.
Pawlowski, J.: Introduction to the molecular systematics of foraminifera, Micropaleontology, 46, 1–12, 2000.
Pawlowski, J. and Holzmann, M.: Diversity and geographic distribution of benthic foraminifera: a molecular perspective, Biodivers. Conserv., 17, 317–328, 2008.
Pawlowski, J. and Holzmann, M.: A plea for DNA barcoding of foraminifera, J. Foram. Res., 44, 62–67, 2014.
Pedersen, T. F. and Price, N. B.: The geochemistry of manganese carbonate in Panama Basin sediments, Geochim. Cosmochim. Ac., 46, 59–68, 1982.
Petersen, J., Riedel, B., Barras, C., Pays, O., Guihéneuf, A., Mabilleau, G., Schweizer, M., Meysman, F. J. R., and Jorissen, F. J.: Improved methodology for measuring pore patterns in the benthic foraminiferal genus Ammonia, Mar. Micropaleontol., 128, 1–13, 2016.
Petersen, J., Barras, C., Bézos, A., La, C., de Nooijer, L. J., Meysman, F. J. R., Mouret, A., Slomp, C. P., and Jorissen, F. J.: Mn∕Ca intra- and inter-test variability in the benthic foraminifer Ammonia tepida, Biogeosciences, 15, 331–348, https://doi.org/10.5194/bg-15-331-2018, 2018.
Pierrot, D., Lewis, E., Wallace, D. W. R. (Eds.): MS Excel program developed for CO2 system calculations, ORNL/CDIAC-105, Carbon Dioxide Inf. Anal. Cent., Oak Ridge Natl. Lab, US Dep. of Energy, Oak Ridge, Tenn., 2006.
Pillet, L., Voltski, I., Korsun, S., and Pawlowski, J.: Molecular phylogeny of Elphidiidae (foraminifera), Mar. Micropaleontol., 103, 1–14, 2013.
Platon, E., Sen Gupta, B. K., Rabalais, N. N., and Turner, R. E.: Effect of seasonal hypoxia on the benthic foraminiferal community of the Louisiana inner continental shelf: The 20th century record, Mar. Micropaleontol., 54, 263–283, 2005.
Polovodova, I. and Schönfeld, J.: Foraminiferal test abnormalities in the western Baltic Sea, J. Foram. Res., 38, 318–336, 2008.
Polyak, L., Stanovoy, V., and Lubinski, D. J.: Stable isotopes in benthic foraminiferal calcite from a river-influenced Arctic marine environment, Kara and Pechora Seas, Paleoceanography, 18, 1003, https://doi.org/10.1029/2001PA000752, 2003.
Punning, J.-M., Martma, T., Kessel, H., and Vaikmäe, R.: The isotopic composition of oxygen and carbon in the subfossil mollusc shells of the Baltic Sea as an indicator of palaeosalinity, Boreas, 17, 27–31, 1988.
Raitzsch, M., Kuhnert, H., Groeneveld, J., and Bickert, T.: Benthic foraminifer Mg∕Ca anomalies in South Atlantic core top sediments and their implications for paleothermometry, Geochem. Geophys. Geosys., 9, Q05010, https://doi.org/10.1029/2007GC001788, 2008.
Raitzsch, M., Dueñas-Bohórquez, A., Reichart, G.-J., de Nooijer, L. J., and Bickert, T.: Incorporation of Mg and Sr in calcite of cultured benthic foraminifera: impact of calcium concentration and associated calcite saturation state, Biogeosciences, 7, 869–881, https://doi.org/10.5194/bg-7-869-2010, 2010.
Raitzsch, M., Hathorne, E. C., Kuhnert, H., Groeneveld, J., and Bickert, T.: Modern and late Pleistocene B∕Ca ratios of the benthic foraminifer Planulina wuellerstorfi determined with laser ablation ICP-MS, Geology, 39, 1039–1042, https://doi.org/10.1130/G32009.1, 2011.
Regenberg, M., Nürnberg, D., Steph, S., Groeneveld, J., Garbe-Schönberg, D., Tiedemann, R., and Dullo, W.: Assessing the effect of dissolution on planktonic foraminiferal Mg/Ca ratios: Evidence from Caribbean core tops, Geochem. Geophys. Geosyst., 7, Q07P15, https://doi.org/10.1029/2005GC001019, 2006.
Roberts, A., Austin, W. E. N., Evans, K., Bird, C., Schweizer, M., and Darling, K.: A new integrated approach to taxonomy: the fusion of molecular and morphological systematics with type material in benthic foraminifera, PLoS One, 11, e0158754, https://doi.org/10.1371/journal.pone.0158754, 2016.
Sadekov, A. Y., Eggins, S. M., and De Deckker, P.: Characterization of Mg∕Ca distributions in planktonic foraminifera species by electron microprobe mapping, Geochem. Geophys. Geosys., 6, Q12P06, https://doi.org/10.1029/2005GC000973, 2005.
Salminen, R. (Ed.): Geochemical Atlas of Europe. Part 1: Background information, methodology and maps, Geological Survey of Finland, Espoo, 526 pp., 2005.
Schmiedl, G., Pfeilsticker, M., Hemleben, Ch., and Mackensen, A.: Environmental and biological effects on the stable isotope composition of recent deep-sea benthic foraminifera from the western Mediterranean Sea, Mar. Micropaleontol., 51, 129–152, 2004.
Scholz, F., Hensen, C., Noffke, A., Rohde, A., Liebetrau, V., and Wallmann, K.: Early diagenesis of redox-sensitive trace metals in the Peru upwelling area – response to ENSO-related oxygen fluctuations in the water column, Geochim. Cosmochim. Ac., 75, 7257–7276, 2011.
Schönfeld, J. and Numberger, L.: The benthic foraminiferal response to the 2004 spring bloom in the western Baltic Sea, Mar. Micropaleontol., 65, 78–95, 2007.
Schweizer, M.: How long after death is DNA preserved in situ in intertidal foraminifera?, J. Micropal., 34, 217–219, 2015.
Schweizer, M., Pawlowski, J., Duijnstee, I. A. P., Kouwenhoven, T. J., and van der Zwaan, G. J.: Molecular phylogeny of the foraminiferal genus Uvigerina based on ribosomal DNA sequences, Mar. Micropaleontol., 57, 51–67, 2005.
Schweizer, M., Pawlowski, J., Kouwenhoven, T. J., Guiard, J., and van der Zwaan, B.: Molecular phylogeny of Rotaliida (Foraminifera) based on complete small subunit rDNA sequences, Mar. Micropaleontol., 66, 233–246, 2008.
Schweizer, M., Pawlowski, J., Kouwenhoven, T., and van der Zwaan, B.: Molecular phylogeny of common cibicidids and related Rotaliida (Foraminifera) based on small subunit rDNA sequences, J. Foram. Res., 39, 300–315, 2009.
Schweizer, M., Jorissen, F., and Geslin, E.: Contributions of molecular phylogenetics to foraminiferal taxonomy: General overview and an example with Pseudoeponides falsobeccarii Rouvillois, 1974, C. R. Palevol., 10, 95–105, 2011a.
Schweizer, M., Polovodova, I., Nikulina, A., and Schönfeld, J.: Molecular identification of Ammonia and Elphidium species (Foraminifera, Rotaliida) from the Kiel Fjord (SW Baltic Sea) with rDNA sequences, Helgoland Mar. Res., 65, 1–10, 2011b.
Segev, E. and Erez, J.: Effect of Mg∕Ca ratio in seawater on shell composition in shallow benthic foraminifera, Geochem. Geophys. Geosys., 7, Q02P09, https://doi.org/10.1029/2005GC000969, 2006.
Skirbekk, K., Hald, M., Marchitto, T. M., Junttila, J., Kristensen, D. K., and Sörensen, S. A.: Benthic foraminiferal growth seasons implied from Mg∕Ca-temperature correlations for three Arctic species, Geochem. Geophys. Geosys., 17, 4684–4704, https://doi.org/10.1002/2016GC006505, 2016.
Tappan, H. and Loeblich, A. R.: Foraminiferal Evolution, Diversification, and Extinction, J. Paleontology, 62, 695–714, 1988.
Toyofuku, T., Suzuki, M., Suga, H., Sakai, S., Suzuki, A., Ishikawa, T., De Nooijer, L. J., Schiebel, R., Kawahata, H., and Kitazato, H.: Mg∕Ca and δ18O in the brackish shallow-water benthic foraminifer Ammonia beccarii, Mar. Micropaleontol., 78, 113–120, 2011.
Tribovillard, N., Algeo, T. J., Lyons, T., and Riboulleau, A.: Trace metals as paleoredox and paleoproductivity proxies: An update, Chem. Geol., 232, 12–32, 2006.
Turner, D. R., Dickson, A. G., and Whitfield, M.: Water-rock partition coefficients and the composition of natural waters – a reassessment, Mar. Chem., 9, 211–218, 1980.
Van Helmond, N. A. G. M., Krupinski, N. Q., Lougheed, B. C., Obrochta, S. P., Andrén, T., and Slomp, C. P.: Seasonal hypoxia was a natural feature of the coastal zone in the Little Belt, Denmark, during the past 8 ka, Mar. Geol., 387, 45–57, https://doi.org/10.1016/j.margeo.2017.03.008, 2017.
Weber, A. A.-T. and Pawlowski, J.: Wide occurrence of SSU rDNA intragenomic polymorphism in foraminifera and its implications for molecular species identification, Protist, 165, 645–661, 2014.
Weldeab, S., Lea, D. W., Schneider, R. R., and Andersen, N.: 155,000 years of West African monsoon and ocean thermal evolution, Science, 316, 1303–1307, 2007.
White, T. J., Bruns, T., Lee, S. J. W. T., and Taylor, J. W.: Amplification and direct sequencing of fungal ribosomal RNA genes for phylogenetics, PCR protocols: a Guide to Methods and Applications, 18, 315–322, 1990.
Widerlund, A. and Andersson, P. S.: Late Holocene freshening of the Baltic Sea derived from high-resolution strontium isotope analyses of mollusk shells, Geology, 39, 187–190, 2011.
Wit, J. C., de Nooijer, L. J., Barras, C., Jorissen, F. J., and Reichart, G. J.: A reappraisal of the vital effect in cultured benthic foraminifer Bulimina marginata on Mg∕Ca values: assessing temperature uncertainty relationships, Biogeosciences, 9, 3693–3704, https://doi.org/10.5194/bg-9-3693-2012, 2012.
Zillén, L., Conley, D. J., Andrén, T., Andrén, E., and Björck, S.: Past occurrences of hypoxia in the Baltic Sea and the role of climate variability, environmental change and human impact, Earth-Sci. Rev., 91, 77–92, 2008.